Understanding DNA to mRNA Translation Process
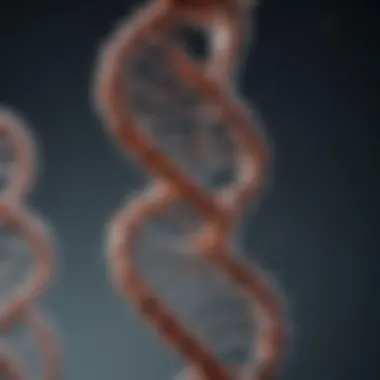
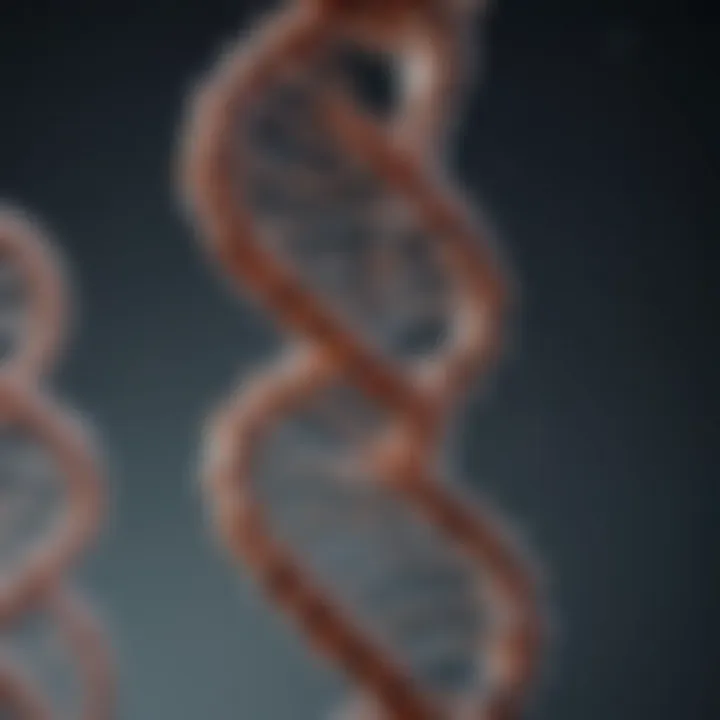
Intro
The process of translating DNA into mRNA is a fundamental mechanism underlying genetic expression. This intricate system ensures that the information held in the DNA is accurately transcribed into a format that cells can utilize to produce proteins. Understanding this transformation is essential for grasping how genetic information translates into biological functions.
In this article, we will dissect this multifaceted process, examining the structural components involved, the stages of transcription, and the roles of various molecular entities. We will also delve into current research avenues that explore and expand our grasp of this essential biological process.
Key Concepts
Definition of the Main Idea
DNA transcription into mRNA is a crucial step in the central dogma of molecular biology. Here, DNA serves as a template for synthesizing messenger RNA. This mRNA then carries the genetic blueprint from the nucleus to the ribosomes, where proteins are synthesized. The significance of this process cannot be understated; it is at the core of cellular functions and ultimately dictates an organism's phenotype.
Overview of Scientific Principles
DNA and mRNA, while both nucleic acids, have distinct structures and functionalities. DNA, which is double-stranded and helical, consists of deoxyribonucleotides containing a phosphate group, a sugar, and nitrogenous bases (adenine, thymine, cytosine, and guanine). Conversely, mRNA is typically single-stranded and composed of ribonucleotides; its base composition includes adenine, uracil, cytosine, and guanine.
The transcription process involves several critical stages:
- Initiation: RNA polymerase binds to the promoter region of the DNA, unwinding the DNA strands to access the template strand.
- Elongation: RNA polymerase synthesizes the mRNA strand by adding complementary nucleotides in the 5' to 3' direction.
- Termination: Transcription continues until RNA polymerase encounters a termination signal, resulting in the release of the newly formed mRNA strand.
Transcription is influenced by various factors, including transcription factors that either promote or repress gene expression. This regulatory mechanism highlights the precision required in gene expression.
Current Research Trends
Recent Studies and Findings
Recent research demonstrates a growing understanding of how specific transcription factors interact with DNA to influence mRNA synthesis. Studies have shown that epigenetic modifications, such as methylation, can affect the efficiency of transcription. These findings have profound implications for understanding diseases like cancer, where alterations in gene expression patterns play a critical role.
Significant Breakthroughs in the Field
Among the notable advancements is the development of CRISPR technology. This tool allows for targeted modifications of DNA, impacting transcription and consequently mRNA production. Such techniques can elucidate complex biological systems and pave the way for potential therapeutic applications.
"The understanding of mRNA synthesis and regulation opens doors to innovative treatments, particularly in genetic disorders and cancer therapies."
"The understanding of mRNA synthesis and regulation opens doors to innovative treatments, particularly in genetic disorders and cancer therapies."
In summary, comprehending how DNA translates to mRNA provides insight into fundamental biological processes, advancing both our scientific knowledge and practical applications in fields like genetic engineering and medicine.
Prelude to Molecular Biology
In the field of biology, molecular biology holds a place of paramount importance. It serves as a bridge connecting various biological disciplines. This section aims to highlight not just the foundational concepts, but also the relevance of molecular biology in understanding how genetic information is expressed and regulated. It provides the framework from which we can explore DNA and RNA's roles in protein synthesis, ultimately illuminating the pathways of life.
Defining DNA and RNA
DNA, or deoxyribonucleic acid, is the hereditary material found in nearly all living organisms. It contains the genetic blueprint for the development, functioning, growth, and reproduction of these organisms. Structurally, DNA is composed of two long strands forming a double helix, made up of nucleotides. Each nucleotide consists of a phosphate group, a sugar molecule, and one of four nitrogenous bases: adenine, thymine, cytosine, or guanine.
RNA, or ribonucleic acid, is typically single-stranded and plays several roles in the cell. It is primarily involved in coding, decoding, regulation, and expression of genes. The nucleotides that compose RNA contain ribose sugar and base uracil, instead of thymine. Understanding the distinctions between these two nucleic acids is fundamental. Their different structures enable them to perform diverse and specific roles in molecular biology.
The Central Dogma of Molecular Biology
The Central Dogma of Molecular Biology encapsulates the flow of genetic information within a biological system. This concept describes the process by which DNA is transcribed into RNA, which is then translated into proteins. Proteins are vital macromolecules that perform countless functions within cells.
Key elements of the Central Dogma include:
- DNA Replication: Before transcription can take place, DNA must replicate itself during cell division, ensuring that genetic information is passed on.
- Transcription: The information encoded in DNA is transcribed to produce messenger RNA (mRNA), which carries the necessary instructions for protein synthesis.
- Translation: The mRNA is then translated by ribosomes into a specific amino acid sequence, forming proteins that perform various cellular tasks.
The Central Dogma is pivotal, as it illustrates not only the sequence of events but also the functional importance of each molecule involved in the flow of genetic information.
The Central Dogma is pivotal, as it illustrates not only the sequence of events but also the functional importance of each molecule involved in the flow of genetic information.
In summary, understanding molecular biology is critical for grasping how life’s complex processes operate at the molecular level. This foundation sets the stage for deeper exploration of DNA to mRNA translation throughout the article.
Chemical Structure of DNA
The understanding of the chemical structure of DNA is paramount in the study of molecular biology and genetics. DNA, or deoxyribonucleic acid, serves as the blueprint for all biological life and allows the storage and transmission of genetic information across generations. By examining its structure, we can appreciate the unique properties that enable DNA to perform its critical functions in the cellular environment. Key aspects of this structure influence not only how genetic information is coded but also how it is replicated and translated into proteins.
Nucleotide Composition
At the core of DNA’s structure are nucleotides, the building blocks of DNA. Each nucleotide consists of three components: a phosphate group, a sugar molecule known as deoxyribose, and one of four nitrogenous bases, which are adenine, thymine, cytosine, and guanine. It is the sequence of these nitrogenous bases that encodes genetic information.
- Phosphate Group: This component links the nucleotides together, forming the backbone of the DNA strand.
- Deoxyribose Sugar: This sugar is key in differentiating DNA from RNA, contributing to the stability of DNA’s structure.
- Nitrogenous Bases: The specificity in base pairing (adenine with thymine and cytosine with guanine) is essential for replication and transcription.
The variable arrangement of these nucleotides creates a unique sequence, determining the specific instructions for synthesizing proteins and regulating cellular functions. The diversity in nucleotide sequences is what encapsulates the distinct characteristics of different organisms.
Double Helix Formation
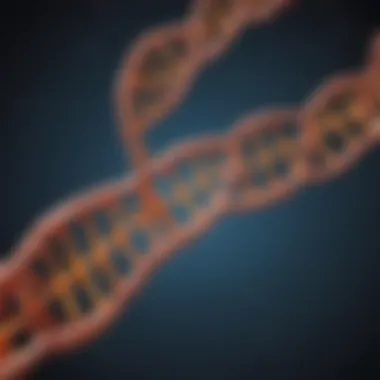
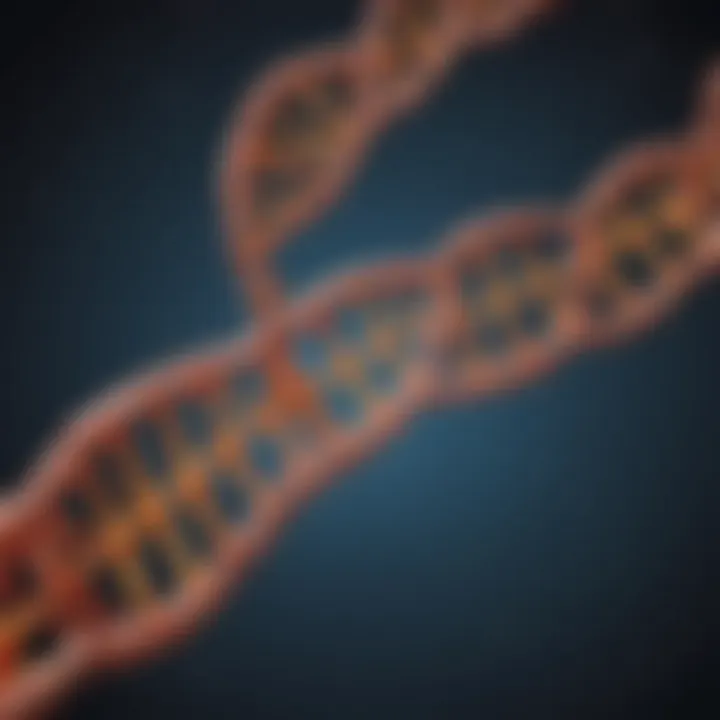
The iconic double helix formation of DNA is another element crucial to its functionality. This structure arises when two strands of DNA intertwine, with the nitrogenous bases facing inward and bonded together by hydrogen bonds. The double helix configuration allows for the stability of genetic information, protecting the information encoded within the bases.
Key points about the double helix:
- Antiparallel Orientation: The two strands run in opposite directions, which is vital for the replication process.
- Base Pairing: Complementary base pairing ensures accurate replication and transcription, reducing the chances of errors that could lead to mutations.
- Major and Minor Grooves: The twisting of the double helix creates major and minor grooves, which play important roles in DNA-protein interactions, allowing transcription factors to access specific sequences.
Understanding the structural characteristics of DNA not only highlights its role in genetics but is also crucial for applications in biotechnology, such as gene editing and synthetic biology.
Understanding the structural characteristics of DNA not only highlights its role in genetics but is also crucial for applications in biotechnology, such as gene editing and synthetic biology.
Chemical Structure of RNA
RNA, or ribonucleic acid, plays a crucial role in the expression of genetic information and the synthesis of proteins. Understanding its chemical structure is essential for comprehending how it functions within biological systems. The structure of RNA is inherently linked to its various roles, making it a fundamental aspect of molecular biology.
The primary unit of RNA is the nucleotide, which consists of three components: a ribose sugar, a phosphate group, and a nitrogenous base. The ribose sugar is integral, contrasting with the deoxyribose found in DNA. This slight change has significant implications for stability and functionality.
Differences Between DNA and RNA
- Sugar Structure: The presence of ribose in RNA includes an -OH group on the second carbon, making it more reactive than DNA, which contains deoxyribose, lacking that -OH group.
- Strands: RNA is generally single-stranded, which allows it to fold into various structures. In contrast, DNA is typically double-stranded, forming a stable double helix.
- Base Composition: RNA contains uracil instead of thymine. This difference affects how RNA interacts during transcription and translation processes.
- Functionality: RNA is versatile. It can be mRNA, tRNA, or rRNA, each serving distinct functions in protein synthesis, while DNA primarily serves as long-term genetic storage.
Understanding these differences is critical for grasping the operational mechanics of cells and the biological significance of RNA.
Types of RNA in Protein Synthesis
The role of RNA in protein synthesis shows the practical application of its structure. There are several types of RNA that facilitate this process:
- Messenger RNA (mRNA): It carries genetic information from DNA to ribosomes, where proteins are synthesized. mRNA is linear and encodes specific proteins based on the sequence of nucleotides.
- Transfer RNA (tRNA): tRNA transports amino acids to the ribosome during protein synthesis. Each molecule of tRNA is charged with a specific amino acid and matches it to the corresponding codon on the mRNA.
- Ribosomal RNA (rRNA): This type combines with proteins to form ribosomes. rRNA is crucial for ensuring the accuracy and efficiency of protein synthesis by facilitating the binding of mRNA and tRNA.
The structural characteristics of RNA, including its single-strandedness and types of nitrogenous bases, are essential for its diverse roles in cellular processes.
The structural characteristics of RNA, including its single-strandedness and types of nitrogenous bases, are essential for its diverse roles in cellular processes.
In summary, the chemical structure of RNA, with its unique features compared to DNA, underpins its functionality in the life of a cell. Understanding these aspects is essential for appreciating the broader processes of transcription and translation, ultimately driving the synthesis of proteins vital for life.
Transcription Mechanism
Transcription is an essential process in molecular biology, bridging the gap between DNA and RNA. The transcription mechanism allows for the transfer of genetic information from DNA to messenger RNA (mRNA), which plays a crucial role in protein synthesis. Understanding this mechanism is vital, as it underpins various cellular functions and the regulation of gene expression. This section will explore the initiation, elongation, and termination phases of transcription, emphasizing their significance and how they contribute to the overall process of gene regulation.
Initiation of Transcription
The initiation of transcription marks the beginning of mRNA synthesis. This phase involves several key steps and molecular interactions that set the stage for accurate transcription.
First, RNA polymerase, the enzyme responsible for creating RNA from the DNA template, binds to a specific region on the promoter of the DNA. The promoter is a sequence of DNA located adjacent to the gene and serves as the starting point for transcription. Once RNA polymerase is bound, it unwinds a small section of the DNA double helix, exposing the nucleotide sequences of the gene.
During this phase, transcription factors also play a critical role. These proteins assist in the recruitment of RNA polymerase to the promoter and regulate the activity of the transcription machinery. Their presence enables the precise recognition of the transcription start site, ensuring that the correct gene is transcribed.
The initiation phase of transcription is crucial for ensuring that genes are expressed at the right time and in the right amounts.
The initiation phase of transcription is crucial for ensuring that genes are expressed at the right time and in the right amounts.
Elongation Phase
After successful initiation, the elongation phase begins. During this stage, RNA polymerase traverses the DNA template strand, synthesizing a complementary mRNA strand in the 5' to 3' direction. The DNA helix unwinds further as RNA polymerase moves along, allowing for the sequential addition of ribonucleotides to the growing RNA chain.
This phase is characterized by a central mechanism, where RNA polymerase matches RNA nucleotides to their complementary DNA bases. A key factor to note is that RNA synthesis does not require a primer, unlike DNA replication. This feature allows for rapid elongation, as numerous ribonucleotides can be added in quick succession.
Moreover, transcription elongation involves proofreading mechanisms. RNA polymerase has the ability to detect and correct mistakes as it synthesizes the mRNA strand, enhancing the fidelity of the transcription process. The elongation process can be influenced by regulatory elements, such as enhancers and silencers, which may enhance or repress the transcription rates of specific genes.
Termination of Transcription
Termination of transcription is the final phase where the synthesis of mRNA concludes. This step is essential for releasing the newly formed mRNA molecule from the transcription complex. The termination signals are typically encoded within the DNA sequence itself and involve specific termination signals recognized by RNA polymerase.
One common mechanism for termination involves the formation of a hairpin structure in the growing mRNA transcript. This structure causes the RNA polymerase to pause and eventually disassociate from the DNA template, effectively ending transcription.
In some cases, specific proteins known as termination factors aid in this process. Once termination is accomplished, the mRNA strand is released for subsequent processing, which includes capping, polyadenylation, and splicing. This ensures that the mRNA is ready for translation into proteins.
By understanding the transcription mechanism, researchers can gain insights into gene regulation, as well as potential implications for biotechnology and medicine.
Role of RNA Polymerase
RNA polymerase is a crucial enzyme in the process of transcription, the first step in the DNA to mRNA translation pathway. Understanding its role allows us to appreciate how genetic information is expressed and how various cellular mechanisms are orchestrated. The enzyme enables the synthesis of RNA from a DNA template, a process fundamental for producing messanger RNA, ribosomal RNA, and transfer RNA, which are essential for protein synthesis.
Function of RNA Polymerase
RNA polymerase carries out several key functions during transcription:
- Initiation: The enzyme binds to the promoter region of a gene, which signals the start of transcription.
- Elongation: Once bound, RNA polymerase unwinds the DNA strands, creating a template strand for RNA synthesis. It assembles ribonucleotides in a specific order complementary to the DNA template.
- Termination: RNA polymerase recognizes signals in the DNA that indicate the end of the gene. Upon reaching this point, it detaches from the DNA, releasing the newly synthesized mRNA.
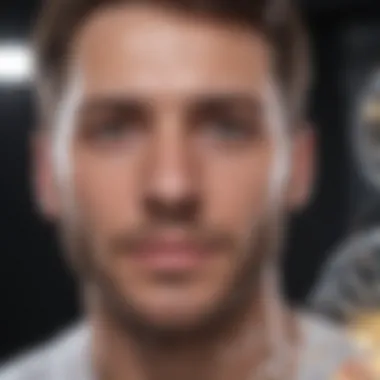
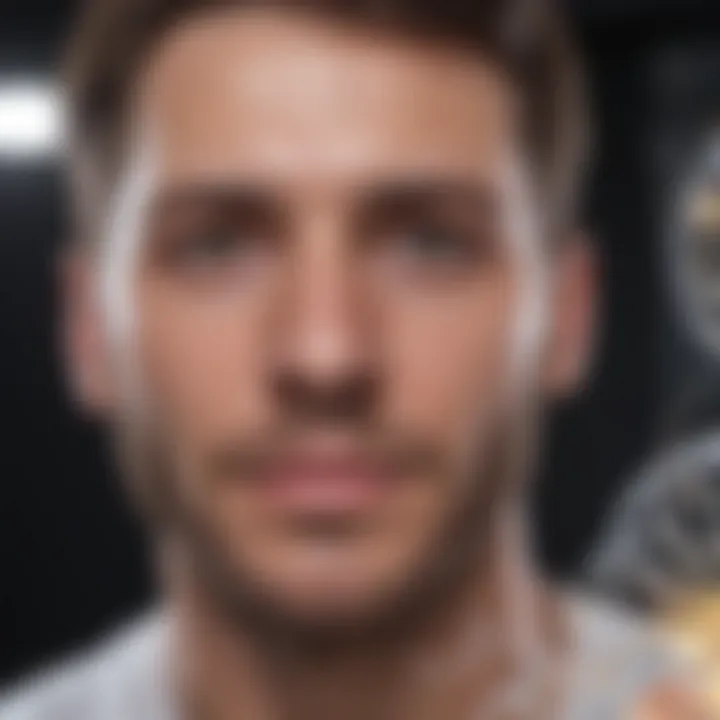
This enzyme operates with a high degree of specificity and efficiency, ensuring that RNA strands are accurately produced. The accuracy of transcription is vital; errors can lead to the production of faulty proteins, contributing to various diseases.
Types of RNA Polymerases
In eukaryotes, there are three main types of RNA polymerases, each with distinct roles in gene expression:
- RNA Polymerase I: This enzyme is primarily responsible for synthesizing ribosomal RNA (rRNA), which is a component of ribosomes. Ribosomes are essential for protein synthesis.
- RNA Polymerase II: This polymerase transcribes mRNA and some snRNA (small nuclear RNA). It is the most studied of the three, due to its pivotal role in synthesizing the mRNA that encodes proteins.
- RNA Polymerase III: This type synthesizes tRNA (transfer RNA) and other small RNAs. tRNA is crucial for translating mRNA into proteins during the process of translation.
Understanding the different functions and types of RNA polymerases helps to clarify their place in both basic biology and biotechnology.
"The precision and functionality of RNA polymerases are fundamental to the integrity of gene expression, impacting everything from cellular function to organismal development."
"The precision and functionality of RNA polymerases are fundamental to the integrity of gene expression, impacting everything from cellular function to organismal development."
In summary, RNA polymerases play an irreplaceable role in transcription, influencing how our genetic information is accessed and utilized. Grasping their functions and types enables deeper insights into molecular genetics and potential areas for biotechnological advancements.
Post-Transcriptional Modifications
Post-transcriptional modifications are crucial in the journey from DNA to mRNA, marking a significant phase in the expression of genetic information. These modifications occur after the initial transcription of mRNA and are integral to ensuring that the mRNA molecule is stable, translatable, and functional within the cell. By refining the mRNA transcript, these processes play an essential role in the regulation of gene expression and have profound implications in various biological contexts, including development, differentiation, and response to environmental signals.
In summary, post-transcriptional modifications enhance the quality and efficiency of mRNA, affecting how genes are expressed. Below, we will dive deeper into two main aspects: Capping and Polyadenylation, followed by RNA Splicing.
Capping and Polyadenylation
Capping and polyadenylation are among the first steps in post-transcriptional modification. The addition of a 5' cap to the mRNA provides several benefits. This cap consists of a modified guanine nucleotide that protects the mRNA from degradation by exonucleases. It also serves as a crucial signal for the mRNA's recognition by ribosomes during translation.
On the other end, polyadenylation involves the addition of a long stretch of adenine nucleotides, commonly referred to as the poly-A tail, to the 3' end of the mRNA. This modification contributes to the stability of the mRNA molecule, facilitating its export from the nucleus into the cytoplasm. Additionally, the poly-A tail encourages efficient translation and contributes to the regulation of the mRNA's lifespan in the cytoplasm, further controlling gene expression.
Some key points regarding capping and polyadenylation include:
- Protection from degradation: The 5' cap and poly-A tail prevent the rapid degradation of mRNA by cellular enzymes.
- Translation initiation: Both modifications are necessary for ribosome recognition and initiation of translation.
- Regulation of gene expression: The length of the poly-A tail can influence the stability and translatability of mRNA transcripts.
Overall, these modifications ensure the proper functioning of mRNA and represent vital regulatory mechanisms in the control of gene expression.
RNA Splicing
RNA splicing is another significant post-transcriptional modification that involves the removal of non-coding regions (introns) from the pre-mRNA transcript. The coding regions, known as exons, are then joined together to form a continuous sequence that will be translated into a protein. This process is essential because, in many genes, the coding sequence is not continuous; instead, it is interrupted by introns that need to be excised.
The splicing process is carried out by a complex known as the spliceosome, which consists of small nuclear RNAs (snRNAs) and protein components. This complex identifies specific sequences at the intron-exon boundaries, facilitating accurate cuts and reintegration of the remaining exons. The ability to vary how exons are combined during splicing leads to the phenomenon of alternative splicing, enabling a single gene to produce multiple protein variants, thus greatly expanding the diversity of proteins that can be generated from the limited number of genes in the genome.
Key elements of RNA splicing include:
- Intron removal: This keeps the mRNA transcript clean and ensures only coding sequences are translated into protein.
- Exon ligation: Connecting the exons is vital for maintaining the correct reading frame needed for proper translation.
- Alternative splicing: This allows for increased protein diversity and adaptability in response to cellular conditions.
Translation Overview
Translation is a crucial process in molecular biology, where the information encoded in mRNA is transformed into a functional protein. This process is essential for cellular function, growth, and response to environmental changes. By translating mRNA into proteins, cells perform a wide variety of roles, from catalyzing metabolic reactions to forming structural components necessary for maintaining cell integrity.
From mRNA to Protein
The journey from mRNA to protein involves several key steps. Initially, the mRNA, which is synthesized during transcription, exits the nucleus and travels to the ribosome, the cellular machine responsible for protein synthesis. The ribosome reads the sequence of codons in the mRNA, each comprising three nucleotides.
As the ribosome reads these codons, transfer RNA (tRNA) molecules play a vital role. Each tRNA matches a specific codon with the appropriate amino acid it carries. Once the ribosome identifies a codon, the corresponding tRNA molecule binds to it, and the amino acid is added to the growing peptide chain. This process continues until a stop codon is reached, leading to the termination of translation.
The sequence of codons in the mRNA dictates the order in which amino acids are linked, resulting in a unique polypeptide chain. The structure of this protein ultimately dictates its function within the cell or organism, making this step critical for biological processes.
Role of Ribosomes
Ribosomes consist of ribosomal RNA (rRNA) and proteins, forming a highly organized complex essential for translation. They can be found freely floating within the cytoplasm or attached to the endoplasmic reticulum, where they facilitate the synthesis of proteins destined for various cellular locations or extracellular export.
The ribosome has three important sites: the A site (aminoacyl site), the P site (peptidyl site), and the E site (exit site). The A site serves as the entry point for incoming tRNA molecules, while the P site holds the growing peptide chain. Once the amino acid is added and the tRNA moves to the E site, it exits the ribosome.
Ribosomes not only translate the mRNA into proteins but also ensure the accuracy of the process. They contribute to the proofreading mechanism that checks for correct tRNA pairing, minimizing errors in protein synthesis. This quality control is vital, as even a single incorrect amino acid can drastically alter a protein's function.
The precision of the translation process is crucial; even minor mistakes can lead to severe malfunctions in biological functions.
The precision of the translation process is crucial; even minor mistakes can lead to severe malfunctions in biological functions.
Understanding translation not only illuminates fundamental biological processes but also carries significant implications for fields like biotechnology and medicine, as manipulating this process can lead to innovative therapies and treatments.
Applications in Biotechnology
The applications of biotechnology rooted in the understanding of DNA to mRNA translation are extensive and profound. The manipulation of genetic information plays a critical role in various fields, including medicine, agriculture, and environmental science. This section will explore how the principles of molecular biology are applied to create innovative solutions that benefit society and enhance our understanding of life itself.
Gene Therapy Innovations
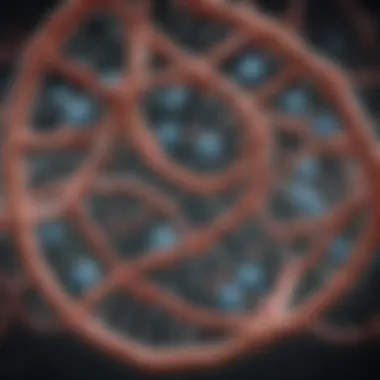
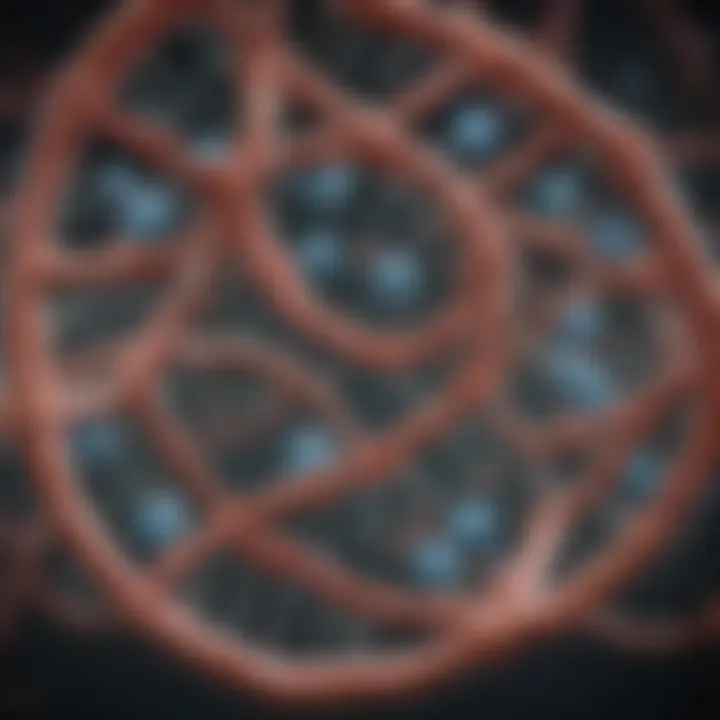
Gene therapy has emerged as a groundbreaking approach to treat genetic disorders. This technique involves modifying genes within an individual’s cells to correct or replace malfunctioning genes. For example, researchers have successfully utilized mRNA technology to deliver corrected genetic sequences to target cells. This can potentially reverse the effects of genetic diseases, such as cystic fibrosis or muscular dystrophy. The advantage of using mRNA in gene therapy is that it can direct the production of functional proteins directly in the patient's cells without altering the patient’s DNA.
Moreover, recent advances in synthetic biology have led to the development of more refined delivery systems for these therapies. Lipid nanoparticles have been instrumental in efficiently delivering mRNA to target tissues. This precision increases treatment efficacy while minimizing side effects, a concern often associated with traditional therapeutic strategies.
The application of mRNA in gene therapy also extends to treatment for cancers and other complex diseases. By instructing cells to produce specific proteins that can target cancer cells, therapeutic mRNA can initiate an immune response against tumors. Such innovations represent a significant leap forward in targeted treatment methodologies.
CRISPR and Genetic Engineering
CRISPR technology has revolutionized genetic engineering, enabling precise modifications of an organism's DNA. By using RNA-guided enzymatic mechanisms, scientists can effectively edit genes at specific locations, offering the potential to correct genetic mutations and enhance beneficial traits.
In the context of mRNA translation, CRISPR can be leveraged to alter mRNA directly. This allows for the regulation of gene expression in a much more controlled manner. For instance, it can be employed to silence malfunctioning genes or even enhance the expression of beneficial genes. The adaptability of CRISPR systems in conjunction with mRNA technology opens a new frontier in biotechnology.
Additionally, CRISPR is being explored in the realm of agriculture, where it can create crops with improved yields and resistance to pests and diseases. This is particularly significant amidst growing concerns about food security and the impact of climate change on agriculture. By engineering plants to withstand environmental stressors, we can ensure a robust food supply for the future.
"Understanding the molecular mechanisms of DNA to mRNA translation enables us to harness the power of biotechnology effectively."
"Understanding the molecular mechanisms of DNA to mRNA translation enables us to harness the power of biotechnology effectively."
In summary, the intersection of mRNA technology and biotechnology is fostering novel approaches in healthcare and agriculture. As research progresses, the potential for innovative solutions continues to expand, demonstrating how the fundamental understanding of molecular biology can translate into practical applications that alleviate suffering and improve quality of life.
Clinical Implications
The clinical implications of DNA to mRNA translation are essential for understanding the broader scope of genetic expressions and its effects on health. This translation process, inherently linked to the synthesis of proteins, contributes to various biological functions and mechanisms. Alterations in this process can lead to significant health issues.
Understanding Genetic Disorders
Genetic disorders arise due to mutations that can affect DNA sequence, thereby impacting mRNA and subsequently protein production. For instance, sickle cell disease is a classic example where a single mutation in the hemoglobin gene leads to the production of an abnormal mRNA transcript. This results in the synthesis of a dysfunctional protein, causing severe health complications.
Key Points about Genetic Disorders:
- Mutation Types: Different mutations, such as point mutations, insertions, and deletions, can disrupt normal mRNA translation.
- Protein Function: The improper translation can affect protein folding or function, leading to various disorders, including cystic fibrosis and muscular dystrophy.
- Diagnosis: Understanding the link between genetic code and clinical manifestation assists in diagnosing genetic diseases.
These insights emphasize that advancing our understanding of mRNA translation can lead to improved diagnostic methods and potential interventions in treating genetic disorders.
mRNA Vaccines and Therapeutics
The emergence of mRNA vaccines marks a significant advancement in therapeutic science. These vaccines utilize the principles of mRNA translation to train the immune system to recognize specific pathogens. Unlike traditional vaccines, mRNA vaccines deliver the genetic information needed for cells to produce an antigen, which stimulates an immune response.
Advancements in mRNA Vaccines:
- Rapid Production: mRNA technology allows for quicker vaccine development. An example is the Pfizer-BioNTech COVID-19 vaccine.
- Precision Medicine: Targeting specific proteins makes mRNA vaccines a vital tool in personalized medicine strategies.
- Broader Applications: mRNA therapeutics are not limited to vaccines. Researchers are exploring their use in cancer treatments by instructing cells to produce protein therapies aimed at cancerous cells.
"The flexibility of mRNA technology could revolutionize how we approach diseases beyond infectious ones, potentially transforming cancer therapy and autoimmune disease treatment."
"The flexibility of mRNA technology could revolutionize how we approach diseases beyond infectious ones, potentially transforming cancer therapy and autoimmune disease treatment."
In summary, the clinical implications stemming from the understanding of DNA to mRNA translation are far-reaching. They bridge the gap between molecular biology and practical applications in medicine, showcasing how genetic information translates into tangible health outcomes.
Current Research Trends
The fast-evolving field of molecular biology brings to light numerous innovations and research pursuits related to DNA and mRNA translation. Understanding these trends is crucial for grasping the broader implications of genetic research and biotechnology. This section elucidates the significance of current research trends, emphasizing their implications on scientific discovery and practical applications.
Innovations in mRNA Technology
Today, mRNA technology has garnered increasing attention due to its pivotal role in vaccine development, cancer therapies, and gene editing. A prime example is the success of mRNA vaccines, such as those developed by Pfizer-BioNTech and Moderna during the COVID-19 pandemic. These vaccines exemplify how synthetic mRNA can instruct cells to produce proteins that stimulate an immune response without introducing live pathogens into the body.
Furthermore, advancements in mRNA modification techniques facilitate better stability and translation efficiency. Researchers are exploring ways to optimize mRNA delivery systems, which is crucial for improving the efficacy of therapies involving mRNA. The impact of these innovations extends far beyond vaccines, with implications for treating genetic disorders and enhancing protein production in various industries.
Future Directions for Study
The future of mRNA technology research appears promising, paving the way for groundbreaking developments. One key area of focus is the integration of artificial intelligence and machine learning to predict mRNA behavior and optimize its design. These computational approaches can accelerate the identification of effective mRNA sequences and regulatory elements.
Moreover, ongoing investigations into employing mRNA for personalized medicine hold great potential. Tailoring mRNA therapies to individual genetic profiles could lead to more effective treatments, especially in oncology, where tumors exhibit significant heterogeneity.
In addition, researchers are delving into the environmental impacts of mRNA production processes. This includes assessing the sustainability of mRNA manufacturing techniques, aiming for scalable and eco-friendly solutions.
"The evolution of mRNA research is a testament to the intersection of biology and technology, providing solutions for some of the greatest health challenges facing society today."
"The evolution of mRNA research is a testament to the intersection of biology and technology, providing solutions for some of the greatest health challenges facing society today."
By fostering collaboration between academia and industry, the prospects for discoveries in mRNA technology are vast, with implications for health care, agriculture, and beyond.
Epilogue
Knowing how DNA translates to mRNA is crucial in understanding biology. This process initiates expression of genes, making it fundamental to all living organisms. mRNA acts as the messenger between genetic information and the proteins that execute various functions in cells.
Reflecting on DNA to mRNA Translation
The transition from DNA to mRNA involves multiple stages. First comes transcription, where the DNA code is copied to form mRNA. This step highlights the precision the cell must maintain. Any errors may lead to malfunctioning proteins, which can have serious consequences. After transcription, post-transcriptional modifications, such as capping and splicing, make the mRNA molecule mature and functional. These processes ensure that the mRNA can effectively convey the genetic blueprint to ribosomes, where translation takes place. This mechanism is also vital for regulating gene expression in response to environmental stimuli.
The Broader Impact on Science and Society
Understanding this translation process has vast implications. In medicine, it opens up pathways for developing therapies, like mRNA vaccines, that have recently gained attention. These solutions have revolutionized approaches to infectious diseases, showcasing the power of this knowledge. Moreover, in biotechnology, innovations such as gene editing tools like CRISPR are based on these fundamental principles. These advancements not only enhance our ability to combat diseases but also raise ethical considerations about genetic manipulation. Thus, the insights gained from studying DNA to mRNA translation resonate across various disciplines, influencing research, health outcomes, and societal norms.