Advancements in Materials Chemistry: A New Era
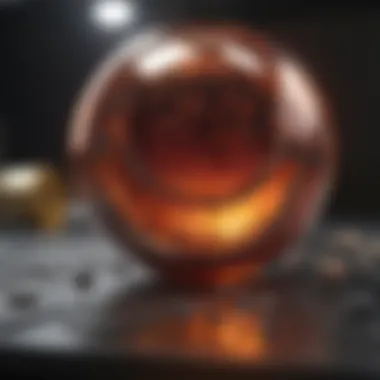
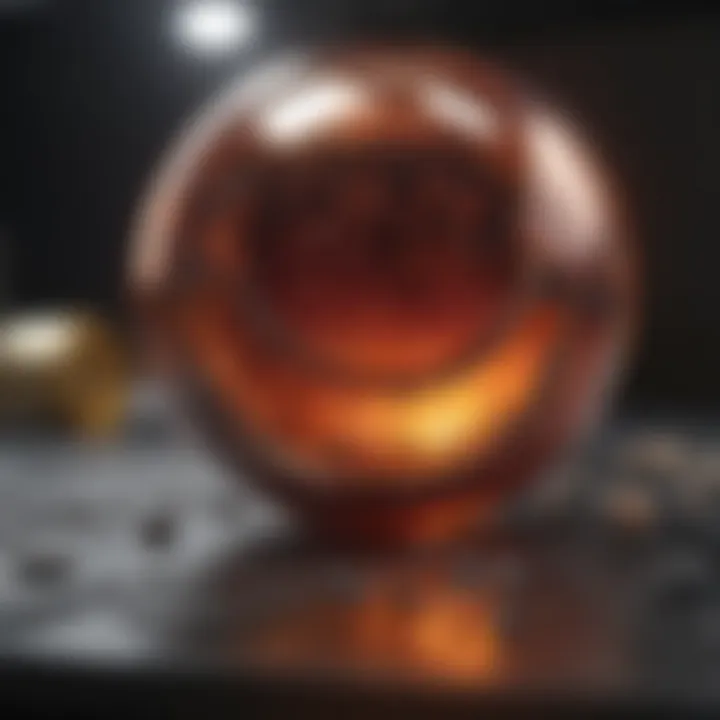
Intro
Materials chemistry stands as an ever-evolving field, bridging gaps between theoretical frameworks and practical applications. It's a domain that synthesizes knowledge from chemistry, physics, engineering, and even biology, pushing the envelope of what’s achievable with material manipulation. From the microscale to the macroscale, the insights garnered from materials chemistry can lead to groundbreaking innovations that ripples through industries such as electronics, energy, and nanotechnology.
The essence of this article serves to encapsulate the advancements, prevailing trends, and future opportunities within this fascinating realm. As we delve into the core concepts, recent research trends, and significant breakthroughs, readers will discover a landscape rich in discovery and promise. It's crucial for students, researchers, educators, and professionals alike to grasp the interconnectedness of theory and experimentation in driving material innovation. By appreciating how these threads weave together, one can better understand how scientists approach challenges and unravel new possibilities.
In a world that often prioritizes speed over substance, the deep dive into the mechanics of materials chemistry encourages a thoughtful exploration and an appreciation of nuance. Each segment of this article is designed to inform and inspire, aiming to shed light on the potential that materials chemistry holds for shaping a sustainable future.
Prolusion to Materials Chemistry
Materials chemistry stands at the confluence of multiple scientific fields, bridging gaps not just within chemistry but also touching on physics, engineering, and materials science. In today’s fast-paced technological landscape, understanding materials chemistry is essential for driving innovation and addressing practical challenges. The study of materials, how they are designed, produced, and applied, plays a critical role in developing new solutions that improve our daily lives and advance various industries.
Definition and Scope
At its core, materials chemistry is the branch of chemistry focused on understanding the relationships between the structure, properties, performance, and applications of materials. This field encompasses a broad scope, including metals, polymers, ceramics, and composites. Each material type serves different functions based on its unique characteristics, which can be manipulated through design and processing.
Key Elements:
- Structure: Examining atomic and molecular arrangements that affect properties.
- Properties: Learning how different conditions, such as temperature and stress, impact material behavior.
- Performance: Testing materials in real-world applications to ensure reliability and safety.
- Applications: Understanding how specific materials can solve particular problems or improve existing solutions.
The relevance of materials chemistry extends beyond the lab; it influences everything from consumer electronics to sustainable energy systems. Emerging technologies, such as high-performance battery materials, smart coatings, and environmental remediation processes, owe their success to advancements in this field.
Historical Context
The journey of materials chemistry dates back centuries, with early advances often intertwined with historical events and technological revolutions. For instance, the development of alloys during the Bronze Age marked a significant leap in materials science, forming the foundation for future innovation.
In the 19th century, the advent of synthetic polymers revolutionized industries, leading to implications in everything from clothing to structural materials. Fast forward to the 20th century, where advancements in understanding atomic structure and bonding facilitated the exploration of nanomaterials and superconductors. This historical framework not only highlights the evolution of materials chemistry but also emphasizes its enduring impact on civilization and industry.
The evolution of materials chemistry is a testament to human ingenuity, illustrating how understanding fundamentals can lead to groundbreaking innovations.
The evolution of materials chemistry is a testament to human ingenuity, illustrating how understanding fundamentals can lead to groundbreaking innovations.
To summarize, the introduction to materials chemistry sets the stage for deeper exploration in this article. From its definition and scope to its rich historical backdrop, the stage is carefully set for a journey into the frontiers of materials innovation.
Key Concepts in Materials Chemistry
Understanding the key concepts in materials chemistry is paramount as these concepts form the foundation upon which innovative materials are developed. This field is not just about understanding the components and structures of materials but also delving into how these aspects influence their properties and potential applications. Knowledge of atomic structure, bonding, and the various properties of materials paves the way for cutting-edge research and applications that can significantly impact various industries, from electronics to renewable energy.
Atomic Structure and Bonding
Covalent Bonds
Covalent bonds are fundamental to many of the materials we encounter every day. They occur when two atoms share electrons, leading to a more stable arrangement. One key characteristic of covalent bonds is their strength, which allows for the creation of resilient materials. This strength is a huge advantage, making covalent bonds a popular choice in constructing complex structures.
A unique aspect of covalent bonds is their directional nature. This means that the shape of the resulting material can be precisely controlled through the arrangement of the bonded atoms. However, this aspect can also be a downside; if not managed properly, it can lead to vulnerabilities in structure under certain stressors, complicating material design.
Ionic Bonds
Ionic bonds involve the transfer of electrons from one atom to another, creating charged ions that attract each other. One of the significant features of ionic bonds is their ability to form crystalline structures, which can be incredibly useful in creating materials such as ceramics and salts. This property offers a beneficial pathway for the development of harder and more durable materials, with applications in everything from construction to electronics.
Nevertheless, ionic bonds are not without their challenges. The rigidity of ionic compounds often leads to brittleness, which can be disadvantageous in applications where flexibility is desired. Understanding these trade-offs is essential for material scientists aiming to fine-tune properties for specific uses.
Metallic Bonds
Metallic bonds are characterized by a 'sea of electrons' that allows for a high degree of conductivity and malleability. This feature makes metallic bonds a preferred choice for applications requiring electrical conductivity and formability, like in electrical wiring and structural components. The delocalized electrons confer significant strength and flexibility to metallic materials, facilitating a vast range of applications across various fields.
However, the very property that allows for these benefits also comes with a wrench in the works. When exposed to certain environments, metals can corrode. Thus, while metallic bonds provide remarkable properties, material scientists must consider environmental stability and degradation in their designs.
Material Properties
Mechanical Properties
Mechanical properties, such as strength, ductility, and hardness, play a crucial role in how materials perform under stress. The importance of these properties cannot be understated; they determine if a material can withstand loads, deformations, or environmental factors in practical applications. A key feature of mechanical properties is that they vary substantially among different materials, leading to tailored solutions for specific applications.
For instance, materials with high tensile strength are invaluable in construction and aerospace industries. However, high strength often comes at the cost of ductility, which can restrict the material’s applications. Balancing these properties is essential for optimizing the performance of materials in real-world scenarios.
Thermal Properties
Thermal properties are vital for assessing how materials respond to temperature changes. The ability to conduct heat and resist thermal expansion can make or break a material's suitability for various applications, especially in electronics and automotive industries, where overheating can be a significant issue. A unique characteristic of thermal properties is their relation to atomic structure; for example, materials with strong covalent bonds often display low thermal conductivity.
Nevertheless, it’s a double-edged sword. Materials that excel in thermal insulation can be limited in their application for heat conduction, highlighting the need for careful selection based on the intended use.
Electrical Properties
Understanding the electrical properties of materials is essential as we venture into an era primarily driven by technology. Conductivity, dielectric strength, and resistivity are characteristics that determine what materials are suitable for electronic devices. A key aspect here is that materials can be designed to enhance specific electrical properties, making them optimal for applications in semiconductors, insulators, and conductors.
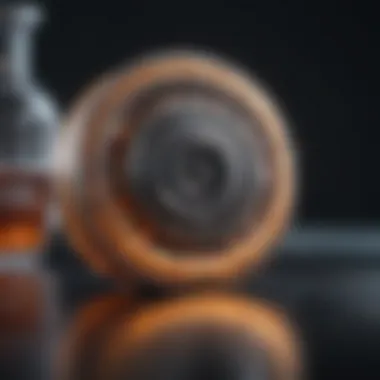
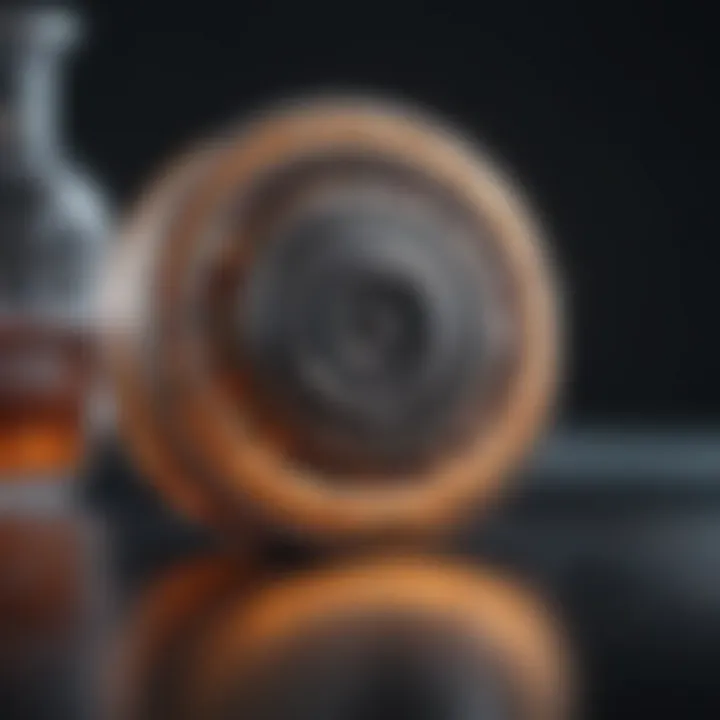
Nonetheless, choosing materials based purely on electrical properties can incur compromises in other areas, such as mechanical stability or thermal resistance. This interconnectedness reinforces the importance of an integrative design approach in materials chemistry.
"The interplay of structure, bonding, and material properties is what drives the innovation in materials chemistry.”
"The interplay of structure, bonding, and material properties is what drives the innovation in materials chemistry.”
In summary, the key concepts in materials chemistry lay the groundwork for the advancements we are witnessing in the field. An awareness of atomic structure and bonding, coupled with an understanding of material properties, empowers researchers and professionals to push the boundaries of what materials can achieve. As we navigate these complex relationships, achieving tailored material solutions becomes not only possible but essential in addressing the challenges of a rapidly evolving technological landscape.
Frontiers in Materials Design
The realm of materials chemistry is expanding rapidly as researchers uncover new dimensions in materials design. This section highlights the pivotal role of innovative approaches in materials design, addressing how these frontiers not only enhance material properties but also resonate with applications across various industries. As the need for specialized materials intensifies, such as those that are light, durable, or responsive to environmental stimuli, the quest for new materials becomes crucial. The development of nanomaterials and smart materials represents significant strides towards achieving these goals, thereby shaping the future landscape of material science.
Nanomaterials
Definition and Types
Nanomaterials are materials with structures sized between 1 and 100 nanometers. Their dimensions allow for unique properties that are markedly different from their bulk counterparts. One of the standout characteristics of nanomaterials is their high surface area to volume ratio, which often leads to increased reactivity and strength. This makes nanomaterials not only a beneficial choice for various applications but also a focal point in the ongoing research within materials chemistry. A defining feature of nanomaterials is their ability to exhibit quantum effects, which can drastically alter electrical, optical, and thermal properties, leading to a range of applications from electronics to medicine. However, their small size can also pose challenges, such as potential toxicity which needs discussion and thorough study.
Synthesis Methods
The synthesis of nanomaterials involves several methods, including top-down and bottom-up approaches. Top-down methods start with larger materials and break them down into nanoscale pieces, while bottom-up techniques build structures from the atomic level up. A key characteristic of these methods is their flexibility in producing materials with various shapes and sizes, making them well-suited for tailored applications. One unique feature of many synthesis methods is their ability to control the properties of the final product through precise manipulation during the manufacturing process. While these methods allow for innovative outcomes, they can also present drawbacks such as high production costs and variabilities in quality.
Applications
Nanomaterials hold transformative potential across various sectors. They are instrumental in medicine for drug delivery systems, enhancing the effectiveness of therapeutics by allowing for targeted delivery. Another major application lies in electronics, where nanomaterials like graphene are revolutionizing conductivity and flexibility of devices. This unique feature of enhanced performance makes them a highly desirable component in cutting-edge technologies. Yet, despite their profile as an advantageous choice, challenges persist primarily surrounding environmental and health impacts that need thorough assessments.
Smart Materials
Characteristics
Smart materials are those that respond to external stimuli, such as temperature, pressure, or electric fields. A notable characteristic of these materials is their inherent ability to alter their properties in reaction to changing conditions. For instance, shape memory alloys can return to a predetermined shape when heated. This dynamic response makes smart materials particularly beneficial in applications that require adaptability. Their unique feature of self-regulation offers an innovative edge, though the complexity of reactions can introduce manufacturing challenges.
Potential Uses
The potential uses of smart materials are vast and diverse, ranging from aerospace and automotive applications to consumer goods. In the medical field, smart materials play a vital role in creating adaptive prosthetics that can better accommodate user needs. Their characteristic responsiveness allows for innovations that can markedly improve performance and user experience. Despite the obvious advantages, the integration of smart materials in products also has its drawbacks, such as a reliance on precise environmental conditions for optimal functionality.
Case Studies
Several case studies underscore the relevance of smart materials in practical applications. For example, the development of self-healing concrete demonstrates how smart materials can enhance structural integrity and longevity. This particular case not only highlights the innovative characteristic of self-repair but also underscores the practical implications for industries plagued by costs associated with repairs and maintenance. By examining various case studies, it's clear that while smart materials offer promising enhancements, limitations often arise in terms of durability, costs, and scalability, requiring ongoing research and optimization.
"The interplay between different materials and their unique properties is redefining our approach to engineering solutions."
"The interplay between different materials and their unique properties is redefining our approach to engineering solutions."
Analytical Techniques in Materials Chemistry
The role of analytical techniques in materials chemistry is akin to the lens through which the intricate world of materials is observed and understood. These techniques provide the essential means to examine, characterize, and manipulate materials at both the atomic and molecular levels. They not only enable the identification of material properties but also contribute significantly to the formulating of hypotheses about the interactions and behaviors of materials under various conditions. As we strive toward innovative applications in electronics, energy storage, and beyond, embracing these analytical tools becomes pivotal in realizing the full potential of new materials.
Spectroscopy
In materials chemistry, spectroscopy serves as a major pillar for investigation and analysis. It provides insight into the relationship between molecular structure and function, revealing the behavior of materials in various environments.
Infrared Spectroscopy
Infrared spectroscopy is a technique that exploits the interaction of infrared radiation with matter to analyze materials. It is particularly valued for its ability to identify functional groups within molecular structures, a key characteristic that helps decipher chemical identities. This is why infrared spectroscopy is often seen as a go-to method for organic compounds in materials chemistry.
The unique feature of infrared spectrometers lies in their non-destructive analysis, allowing researchers to gather information without altering the sample. However, a significant disadvantage is that it may struggle with complex mixtures, making interpretations tricky in some cases. Nonetheless, its affordability and relatively simple setup make it a practical choice for various applications, including polymer analysis and quality control in material production.
Nuclear Magnetic Resonance
Nuclear Magnetic Resonance (NMR) is another powerful analytical method. It capitalizes on the magnetic properties of atomic nuclei, providing detailed information about the molecular structure and dynamics of materials. The key characteristic of NMR that sets it apart is its ability to give insight into the connectivity and environment of specific atoms in a molecular framework.
NMR’s unique feature is its capacity to distinguish between different isotopes, making it highly useful for tracking specific elements within complex samples. However, the requirements for high-end equipment and expertise to analyze the data can be barriers in some laboratories. Regardless, for those equipped with the resources, NMR remains a gold standard in molecular analysis.
Mass Spectrometry
Mass spectrometry (MS) rounds out the spectroscopy techniques vital to material characterization. MS is unmatched in its accuracy when it comes to determining molecular weight and revealing the composition of materials, which is vital when assessing new compounds.
Its defining characteristic is its high sensitivity and resolution, allowing the detection of even minute quantities of substances. One can describe the unique feature of MS as the ability to analyze the ionic fragments of a molecule to infer its structure. However, one downside is that sample preparation can be intricate, making it less user-friendly for rapid assessments. Still, for in-depth analysis, MS is an indispensable tool in the arsenal of materials chemists.
Microscopy
Microscopy techniques are equally vital and enhance our understanding of materials' features at a micro to nano scale. They provide spatial resolution that is critical in exploring surface characteristics and internal structures of materials.
Transmission Electron Microscopy
Transmission Electron Microscopy (TEM) provides a cross-section view of materials by transmitting a beam of electrons through a specimen. The key part of TEM is its incredibly high-resolution imaging, which can achieve near atomic-scale observations. This is crucial for understanding the structural attributes of materials, especially in research involving nanostructured materials.
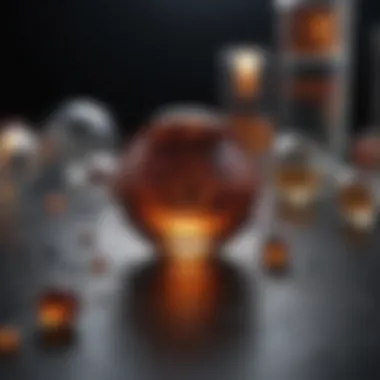
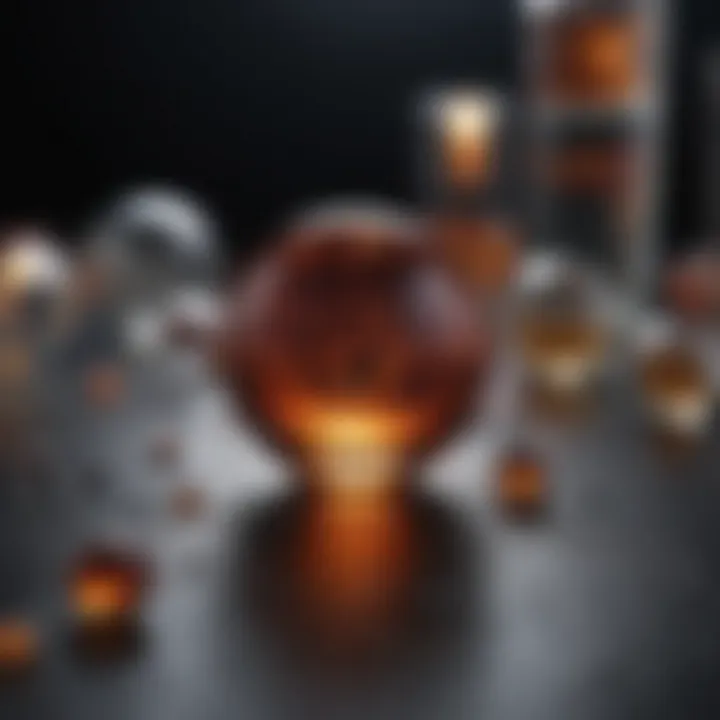
Its unique feature allows for imaging and analyzing materials without extensive modifications, enabling the study of samples in their native states. However, sample preparation can be quite demanding and time-consuming. Nevertheless, for researchers looking to study thin materials or nanoscale phenomena, TEM is unsurpassed.
Scanning Electron Microscopy
On the other hand, Scanning Electron Microscopy (SEM) offers different insights by scanning a focused beam of electrons over a material's surface. The notable characteristic of SEM is its ability to produce three-dimensional images, providing detailed topographical information.
Its unique aspect lies in the relative ease of sample preparation compared to TEM, making it a popular choice among researchers. However, one downside is that SEM cannot give the same level of internal detail as TEM, which means it is often used complementary to other techniques. In material science, SEM serves as an invaluable tool for examining surface structures and interfaces in depth.
Atomic Force Microscopy
Atomic Force Microscopy (AFM) steps in as a technique providing high-resolution, three-dimensional surface profiling. AFM’s key characteristic is that it can visualize surfaces at the atomic level, revolutionizing the way we understand material surfaces and functionalities.
The unique feature of AFM lies in its versatility; it can be conducted in ambient conditions or controlled environments. This flexibility allows investigation across various materials types. However, it can be time-consuming and demanding on the operator's skills, limiting its use in some settings. Regardless, for those engaged in nanotechnology and advanced materials science, AFM is an essential contributor to material characterization.
Emerging Technologies in Materials Chemistry
Emerging technologies in materials chemistry stand at the forefront of revolutionary developments influencing various industries. These innovations not only redefine how we understand materials but also enhance their applications across domains. The shift to cutting-edge techniques signifies a deeper grasp of materials’ behavior and potential, paving the way for improved performance and efficiency in various applications.
Machine Learning in Material Discovery
Data-Driven Approaches
Data-driven approaches have come to the forefront of material discovery, enabling scientists to sift through vast amounts of data swiftly. The key characteristic of this method is its ability to analyze and extract meaningful insights from complex datasets, often using algorithms to identify patterns and correlations. This is particularly beneficial in materials chemistry because it allows for rapid screening of potential materials without the need for exhaustive experimental processes.
The unique feature of data-driven approaches lies in their reliance on existing experimental data to predict the properties of new materials. While this can significantly shorten the development time for new materials, a potential disadvantage is the accuracy of predictions, which may vary based on the quality and quantity of data used.
Predictive Modeling
Predictive modeling serves as a complementary aspect to data-driven approaches, applying statistical techniques to forecast material behaviors and properties. It is widely accepted due to its systematic application in optimizing material performance. The predictive models can assess numerous variables and constraints to find the most suitable materials for specific applications.
One unique feature of predictive modeling is its capability to simulate real-world conditions for materials performance, which helps in making informed decisions before physical prototypes are created. A downside could be the dependency on accurate baseline data and assumptions which, if flawed, may lead to unreliable outputs.
Real-World Examples
Real-world examples of machine learning applications in material discovery illustrate the practicality of these methods. For instance, researchers have successfully developed new polymers and composites for uses in industries ranging from aerospace to healthcare by employing machine learning algorithms. This characteristic of translating theoretical modeling to practical applications adds significant value.
These instances showcase the benefits of machine learning in devising innovative materials. Yet, challenges such as integration with existing workflows and a learning curve for scientists in interpreting machine-generated data remain hurdles to overcome.
3D Printing of Materials
Techniques and Methods
The landscape of manufacturing has transformed with the advent of 3D printing, particularly in materials chemistry. Various techniques like Fused Deposition Modeling and Stereolithography represent just a few methodologies enabling the layering of materials to create intricate designs. The ability to produce highly customized objects on demand emphasizes the significance of this technology in contemporary material applications.
What makes these techniques particularly exciting is their adaptability—materials can be engineered at micro and macro levels, enabling entirely new structures that traditional manufacturing cannot easily produce. However, a challenge that 3D printing faces is the variability in material properties based on the printer's settings and the material composition used.
Applications Across Industries
3D printing presents unique applications across multiple industries, including automotive, aerospace, and healthcare. In the medical realm, for example, custom implants and prosthetics can be quickly tailored to individual patients. This adaptability is a crucial characteristic that highlights 3D printing's potential to revolutionize product customization.
One unique trait of its application is the reduction in material waste, as items are produced layer by layer rather than from a block of material. A potential drawback, however, is that some 3D-printed materials may not yet meet stringent regulations in certain sectors, limiting their use.
Future Perspectives
Looking forward, the prospects of 3D printing and its integration with advanced materials chemistry is vast. The continual improvement in printing techniques and material variety will likely lead to even broader applications and improvements in existing processes. The key characteristic of future perspectives lies in their potential to eliminate barriers in material design and production.
One unique aspect of these perspectives is the exploration into bioprinting, opening doors to new medical applications such as organ printing, which comes with both massive potential and ethical considerations. While the future looks bright, challenges in ensuring the reproducibility and reliability of manufactured objects must continuously be addressed.
Applications of Materials Chemistry in Industry
In the vast ocean of modern science, materials chemistry holds a significant place. Its applications in various industries not only enhance existing technologies but also pave the way for new innovations. From electronics to energy, materials chemistry serves as the backbone for numerous advancements that impact daily life. Understanding its role is essential for researchers and professionals alike, particularly as we face pressing global challenges.
The relevance of materials chemistry in industry cannot be overstated. By optimizing material properties, we can achieve efficiency, durability, and sustainability in applications that touch almost every facet of our lives. This section delves into two pivotal areas where materials chemistry plays a critical role: electronics and optoelectronics, as well as energy storage and conversion.
Electronics and Optoelectronics
As we venture into the world of electronics and optoelectronics, it’s clear that materials chemistry is at the heart of technological advancement. The devices we rely on today, from smartphones to solar panels, depend heavily on innovative materials designed at the molecular level. Advancements in semiconductors, conductive polymers, and nanoscale materials have revolutionized these fields.
- Semiconductors: Traditional silicon has served as the primary material for electronics. However, the rise of materials like graphene and transition metal dichalcogenides offers remarkable improvements in conductivity and flexibility, enabling the development of more efficient and lightweight devices.
- Conductive Polymers: These materials act as both conductors and insulators. They are increasingly used in applications like organic light-emitting diodes (OLEDs), which are found in high-end displays. Their adaptability allows for a new level of creativity in product design.
The push towards sustainable electronics has led to increased interest in biodegradable materials as well. By blending natural and synthetic materials, researchers are actively seeking to address the growing issue of electronic waste.
Energy Storage and Conversion
Shifting our focus to energy storage and conversion, materials chemistry has emerged as a lighthouse guiding us towards more efficient and sustainable energy solutions. As society moves away from fossil fuels, the demand for advanced energy storage systems has skyrocketed. Here, materials chemistry is essential in developing batteries, supercapacitors, and fuel cells.
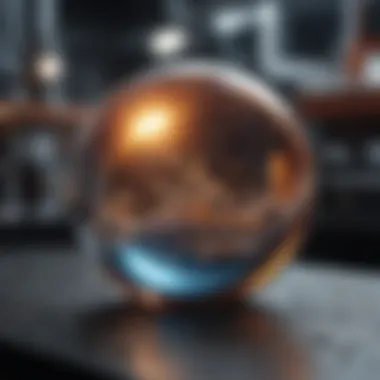
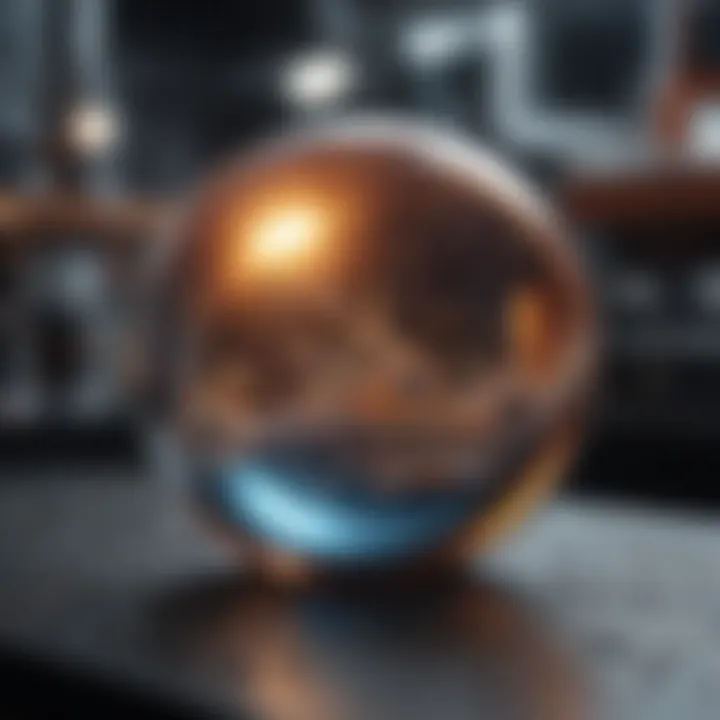
- Batteries: The lithium-ion battery, the workhorse of portable electronics, has transformed how we think about energy storage. Researchers are currently exploring alternative chemistries, such as sodium-ion and solid-state batteries, which promise greater safety and higher energy densities.
- Supercapacitors: These devices bridge the gap between batteries and traditional capacitors, providing rapid charge and discharge capabilities. Advanced carbon-based materials offer substantial improvements in performance, which can lead to more efficient energy management systems.
- Fuel Cells: Employing materials chemistry in catalysts is a game changer. Platinum has been the go-to material, but challenges like cost and scarcity necessitate the search for alternative catalysts. Research into non-precious metal catalysts is promising and may eventually result in more economical fuel cell technologies.
"The future rests on innovative materials that not only improve technological capabilities but also address our planet's environmental challenges."
"The future rests on innovative materials that not only improve technological capabilities but also address our planet's environmental challenges."
Further reading on materials chemistry applications can be explored on Wikipedia or Britannica.
Moreover, discussions around the impact of materials science on social media platforms like Reddit can provide valuable insights.
For more discussions and updates, follow relevant groups on Facebook.
Understanding these applications is just the beginning. With ongoing research and development, future innovations are bound to make our world more connected and sustainable.
Environmental Considerations in Materials Chemistry
In an era when ecological sustainability is gaining traction, understanding the role of materials chemistry within the broader environmental framework is paramount. The production and use of materials often come with environmental implications that cannot be overlooked. As this article explores the innovations in materials chemistry, it’s equally important to address the pressing environmental considerations that accompany these advancements. It becomes increasingly clear that while new materials can provide better performance and efficiency, their production, maintenance, and eventual disposal must also be sustainable. Failing to do so could lead to adverse environmental impacts that counteract the benefits of these innovations.
Sustainability in Material Production
Sustainability in material production is not just a buzzword; it reflects a genuine commitment to reducing carbon footprints, conserving resources, and ensuring that materials can be produced without causing harm to the environment. The traditional approaches to material production often relied heavily on non-renewable resources and energy-intensive processes. But new strategies are emerging. These strategies aim to incorporate renewable sources and minimize waste.
Factors driving this transformation include:
- Use of Renewable Feedstocks: By using sustainable raw materials such as biomass, manufacturers are reducing their dependence on fossil fuels.
- Energy Efficiency: New production techniques focus on using less energy, such as through more efficient catalytic processes.
- Eco-Friendly Chemicals: Substituting harmful chemicals with greener alternatives can minimize pollution during production.
Innovations like bio-based polymers, which are derived from natural sources, are excellent examples of this shift. They not only serve their purpose but also break down more easily, reducing the long-term impact on landfills. Additionally, by aligning with regulations and market preferences for eco-friendly options, companies can position themselves advantageously in the marketplace. This trend indicates a promising route toward achieving sustainable practices in materials chemistry.
Recycling and Material Lifecycle
The life cycle of materials plays a crucial role in their environmental impact. Recycling is no longer an afterthought; it has become a fundamental component of material management systems. The full life cycle entails extraction, production, use, and end-of-life disposal, an area featuring many opportunities for improvement.
Some significant aspects of recycling and material lifecycle include:
- Closed-Loop Recycling: Creating materials that can be recycled indefinitely and used to produce new items. This helps minimize waste and preserve the initial raw materials.
- Lifecycle Assessment (LCA): This methodological tool helps in assessing the environmental impacts associated with all stages of a product's life. Through LCA, researchers can identify potential areas for improvement and optimisation.
- Consumer Awareness: The rising demand for sustainable products is pushing manufacturers to develop systems for easy recycling and eco-friendliness.
"A greener material lifecycle ensures that materials do not just exist to serve a single function but can contribute to a circular economy."
"A greener material lifecycle ensures that materials do not just exist to serve a single function but can contribute to a circular economy."
Fostering a culture of recycling not only decreases the amount of waste that ends up in landfills but also supports the conservation of the resources needed to create new materials. Addressing these questions is vital not only for the industry but for society as a whole. As materials chemistry continues to evolve, placing emphasis on sustainability and recycling will shape its future, ensuring that innovations benefit both human life and the environment.
Interdisciplinary Approaches in Research
In the realm of materials chemistry, the confluence of diverse fields is more than just a trend; it's a necessity. By blending the insights from chemistry, physics, engineering, and even data science, researchers can delve deeper into material properties, unlocking new potential applications. This interdisciplinary synergy not only enhances the pace of innovation but also broadens the problem-solving toolkit available to scientists. Understanding how different disciplines can cooperate enhances the capability to address complex challenges, paving the way for revolutionary breakthroughs in material design.
Collaboration Between Disciplines
Collaboration is the heart of interdisciplinary research. Take a typical project involving nanomaterials; chemists might synthesize novel compounds, while physicists could characterize their electronic properties. When teams come together, they pool their expertise, leading to outcomes that no single discipline could achieve
- Cross-Pollination of Ideas: Each discipline brings unique methodologies and perspectives. A chemist might be aware of new synthesis routes; an engineer may know how to scale these processes efficiently.
- Resource Sharing: Research institutions often have access to advanced equipment and technologies that might be out of reach for individual labs. Sharing these resources can accelerate research timelines.
- Diverse Problem-Solving Approaches: With multiple viewpoints on a problem, solutions are likely more innovative. Moving away from traditional paths often leads to unexpected and fruitful avenues of inquiry.
Research initiatives, such as collaborative grants from scientific foundations, are increasingly advocating for interdisciplinary teams. These grants not only fund the research but also encourage teams to think outside the box, pushing boundaries and discovering new material functionalities.
Research Institutions and Industry Partnerships
The relationship between academic research institutions and industry plays a crucial role in advancing materials chemistry. These partnerships facilitate the translation of theoretical research into practical applications. Through collaborative efforts, valuable knowledge transfer occurs, turning scientific discoveries into commercially viable products.
- Case Studies: Many successful innovations have arisen from such collaborations. For example, the development of lithium-ion batteries benefitted immensely from joint efforts between research labs and tech companies, allowing for more efficient energy storage solutions.
- Curriculum Development: Many institutions tailor their research programs based on industry needs. By working closely with companies, educational institutions can prepare their students for real-world challenges. This dynamism ensures that the next generation of materials chemists is equipped with the skills that industries seek.
- Funding Opportunities: Industry partnerships often come with financial backing, allowing researchers to hold larger-scale experiments or pursue ambitious long-term studies that academic funding may not cover.
"The challenges in materials research today require diverse skill sets and a collaborative spirit that transcends traditional disciplinary boundaries."
"The challenges in materials research today require diverse skill sets and a collaborative spirit that transcends traditional disciplinary boundaries."
Future Directions in Materials Chemistry
Future directions in materials chemistry offer a glimpse into the evolving landscape of this vibrant field. As research and technology blend seamlessly, the importance of predicting advancements and recognizing challenges can't be overstated. These considerations play a key role in shaping the trajectory of materials chemistry, impacting everything from next-generation materials to sustainability efforts.
Predictions for Advancements
In the realm of materials chemistry, making accurate predictions is as critical as the actual experiments in the lab. While some advancements, such as the growth of nanomaterials and smart materials, seem imminent, others might take a longer route due to unforeseen complications. Here are some predictions worth noting:
- Integration of Artificial Intelligence: AI will enhance our ability to design and optimize materials at an unprecedented pace. Algorithms can analyze vast datasets, leading to quicker discoveries in material properties and applications.
- Biomimetic Materials: Nature has engineered materials over millions of years, and mimicking those designs could lead to breakthroughs. For example, the structure of spider silk could inspire new, stronger composites.
- Sustainable Materials: The push towards sustainability will drive the development of eco-friendly materials that are biodegradable or recyclable. Innovations in this area will likely see a rise in bio-based polymers and derived materials.
"The true potential of materials science lies not just in its innovations, but also in its ability to address global challenges through responsible research."
"The true potential of materials science lies not just in its innovations, but also in its ability to address global challenges through responsible research."
These predictions highlight a future where the interplay between technology, environment, and material properties becomes increasingly significant. As ideas transition from theory to practical applications, the relevance of such advancements cannot be understated.
Challenges Ahead
While the future looks promising, the road ahead is not without its potholes. The challenges facing materials chemistry are multifaceted and require a concerted effort from researchers, industries, and policymakers alike. Here are some critical challenges to keep in mind:
- Scalability of Innovations: Even if a new material shows promise in the lab, scaling its production to meet industrial needs is complicated. Finding ways to transition from small-scale prototypes to large-scale manufacturing without sacrificing quality is vital.
- Environmental Impact: While innovating new materials, it’s crucial to assess their environmental footprint. Some materials may have hidden costs that aren’t apparent in initial research phases. Ensuring sustainable practices throughout the lifecycle of materials is a growing concern.
- Interdisciplinary Collaboration: The complexities of modern materials require collaboration across various fields. However, achieving effective communication and coordinated efforts between disciplines remains a hurdle.
In sum, as researchers venture into the future of materials chemistry, they are met with both exhilarating possibilities and daunting obstacles. By understanding the predictions and preparing for challenges, the community can work towards solutions that benefit not only the field itself but society at large.