Understanding the DNA to Protein Process: A Deep Dive
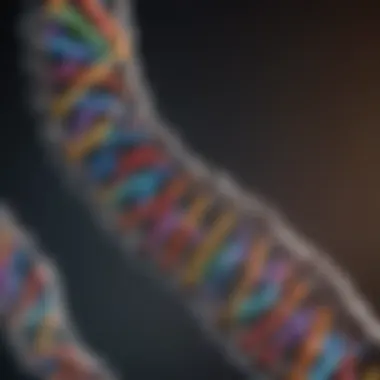
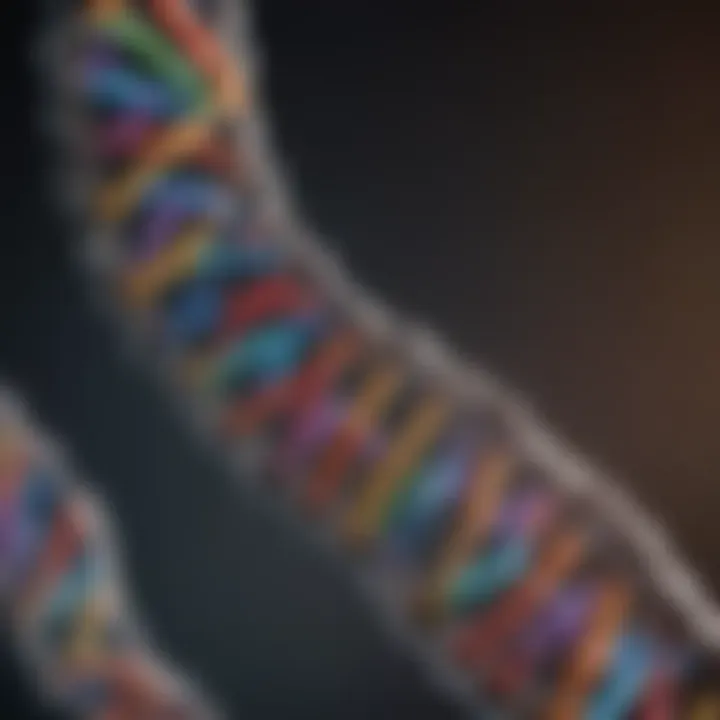
Intro
The process of converting DNA to protein is essential for the functioning of all living organisms. It is a multi-step mechanism that ensures the instructions encoded in our genetic material lead to the synthesis of proteins, the building blocks of life. Proteins are not only structural elements; they are also involved in various biochemical reactions, acting as enzymes, hormones, and signaling molecules. Understanding how DNA is translated into functional proteins gives insight into cellular operations and the biological processes that sustain life.
Key Concepts
Definition of the Main Idea
At its core, the DNA to protein process involves two primary stages: transcription and translation. During transcription, a specific segment of DNA is copied into messenger RNA (mRNA). This mRNA then serves as a template for translation, where it guides the synthesis of proteins on ribosomes with the help of transfer RNA (tRNA).
Overview of Scientific Principles
The structure of DNA is a double helix composed of nucleotides, which contain a phosphate group, a sugar, and nitrogenous bases. There are four bases: adenine (A), thymine (T), cytosine (C), and guanine (G). The sequence of these bases encodes genetic information, dictating the type and order of amino acids in a protein.
Key steps in the process include:
- Transcription: The DNA unwinds and RNA polymerase synthesizes mRNA, complementary to the DNA template strand.
- Translation: Ribosomes read mRNA sequences, and tRNA molecules bring corresponding amino acids together to form polypeptides.
- Post-Translational Modifications: After synthesis, proteins may undergo additional modifications, altering their functionality and activities.
Understanding this process reveals the complexity of gene expression and how cells respond to varying conditions.
Understanding this process reveals the complexity of gene expression and how cells respond to varying conditions.
Current Research Trends
Recent Studies and Findings
Recent advancements in molecular biology have revealed new details about the intricacies of transcription and translation. For example, researchers have discovered how ribosomes can pause during translation, allowing for quality control before protein synthesis completes. Understanding these pauses can uncover the mechanisms underlying diseases that stem from protein misfolding.
Significant Breakthroughs in the Field
Recent breakthroughs include the development of CRISPR technology, which allows for targeted editing of genes. This method has the potential to correct mutations at the DNA level, thereby impacting the protein that is ultimately produced. Likewise, studies involving synthetic biology are creating engineered organisms that can produce custom proteins, which may have applications in medicine and industry.
Continued exploration in this fascinating area of genetics and molecular biology is crucial for advancements in health and disease management. Understanding the nuances of the DNA to protein process paves the way for innovative solutions in biotechnology, drug development, and genetic engineering.
Prelims to the DNA to Protein Process
Overview of Molecular Biology
Molecular biology focuses on the structure and function of the molecules that govern life. It explores critical processes such as the replication of DNA, the synthesis of RNA, and the formation of proteins. This discipline emphasizes how molecular interactions and biochemical reactions facilitate the maintenance and evolution of life. A comprehensive understanding of molecular biology informs researchers about disease mechanisms and the development of new therapeutic approaches.
Importance of Proteins in Biological Systems
Proteins serve as the building blocks of life. They perform a variety of functions that are crucial for the survival and efficiency of biological systems. These include:
- Enzymatic activities that catalyze biochemical reactions.
- Structural support in cells and tissues, such as collagen in connective tissues.
- Transport functions, like hemoglobin in blood that carries oxygen.
- Signal transduction, which helps relay messages within and between cells.
Proteins are vital for nearly every cellular process. Their diverse roles underscores their importance in biological function and health.
Proteins are vital for nearly every cellular process. Their diverse roles underscores their importance in biological function and health.
The significance of proteins cannot be overstated. They are involved in virtually every cellular activity, making it essential to understand how they are synthesized from DNA.
The Structure of DNA
The structure of DNA is central to understanding the entire DNA to protein process. DNA, which stands for deoxyribonucleic acid, contains the genetic blueprint for all living organisms. It plays a critical role in storing and transmitting genetic information. The structural features of DNA allow it to serve this function remarkably well.
Components of DNA
DNA is made up of several key components that interact with each other to form a functional molecule. These components include:
- Nucleotides: The building blocks of DNA. Each nucleotide consists of a phosphate group, a sugar molecule, and a nitrogenous base. The four nitrogenous bases are adenine (A), thymine (T), cytosine (C), and guanine (G).
- Sugar-Phosphate Backbone: The sugar and phosphate groups form a backbone, providing structural support to the DNA molecule. This backbone runs along the length of the DNA strand and is highly stable.
- Nitrogenous Bases: The bases are crucial for encoding genetic information. They pair specifically: adenine with thymine, and cytosine with guanine. These pairs form the rungs of the DNA ladder, important for information transmission.
Understanding these components is vital. It clarifies how genetic information is stored and replicated, influencing various biological processes, including those leading from DNA to protein synthesis.
Double Helix Formation
The double helix structure of DNA is its most notable feature. This configuration was elucidated by James Watson and Francis Crick in 1953. The double helix framework consists of two long strands of nucleotides coiled around each other. Here are some important aspects of this structure:
- Antiparallel Strands: The two strands run in opposite directions, which is essential for replication and transcription. This orientation affects how enzymes read and process the DNA.
- Hydrogen Bonds: The base pairs connect via hydrogen bonds, allowing the strands to separate easily when needed, such as during replication or when RNA is transcribed from DNA.
- Major and Minor Grooves: The winding of the helix creates major and minor grooves. These grooves provide access for proteins and enzymes, facilitating DNA replication and transcription.
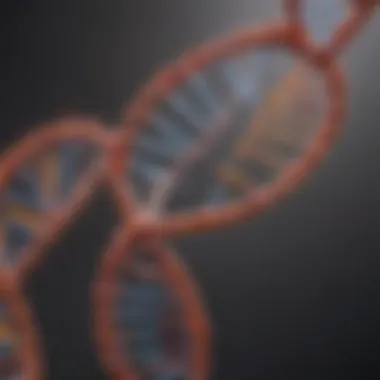
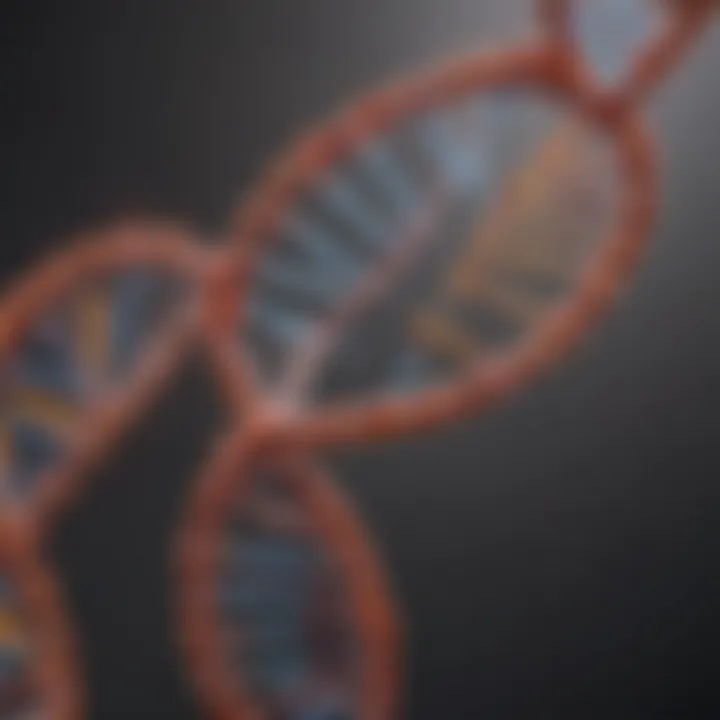
The double helix is not just a stable structure; it is also dynamic. It can twist, untwist, and open up as various cellular processes take place. Its design elegantly supports the critical task of maintaining and expressing genetic information.
"The double helix is a remarkable design that supports the complex functions and processes of life."
"The double helix is a remarkable design that supports the complex functions and processes of life."
In summary, the structure of DNA underpins its role in the flow of genetic information. Each component and feature contributes to its functions, thereby influencing the entire process of how proteins are synthesized from this genetic blueprint.
Transcription: From DNA to RNA
Transcription is the first critical step in the journey from DNA to protein. This process involves converting the genetic information stored in DNA into a complementary RNA sequence. Understanding transcription is vital, as it lays the groundwork for subsequent protein synthesis during translation. The accurate transcription of genes ensures that cells produce the right proteins necessary for various biological functions.
Initiation of Transcription
The initiation phase marks the beginning of transcription. It requires the binding of RNA polymerase to a specific region of DNA known as the promoter. This region contains important signals that direct the RNA polymerase to the correct gene for transcription. The transcription factors also play a crucial role in this stage by helping the RNA polymerase recognize the promoter. Once the transcription complex is formed, the DNA strands unwind and separate, allowing the RNA polymerase to access the template strand. The importance of this initiation phase cannot be overstated, as it determines which genes will be expressed in a given cell type.
Elongation of RNA Strand
After initiation, the elongation phase ensues. During this stage, RNA polymerase synthesizes the RNA strand by adding ribonucleotides complementary to the DNA template strand. This process occurs in the 5’ to 3’ direction, meaning each new nucleotide is added onto the 3’ end of the growing RNA chain. The RNA polymerase moves along the DNA, continuously unwinding the double helix and exposing the gene coding region. This phase is critical, as it determines the length and sequence of the RNA molecule being produced, which has direct implications on the final protein structure.
Termination of Transcription
Termination occurs when RNA polymerase encounters specific signals in the DNA sequence that indicate the end of the gene. This allows the polymerase to disengage from the DNA, releasing the newly synthesized RNA molecule. In eukaryotes, the termination process can be complex, often involving additional protein factors that assist in ending transcription. Understanding this step is essential for recognizing how versatile gene expression is regulated based on cellular needs.
Post-Transcriptional Modifications
After transcription, the RNA undergoes several modifications before it is exported for translation. These modifications are essential for the stability, transport, and function of the RNA.
' Capping
5' capping involves the addition of a modified guanine nucleotide to the 5' end of the RNA transcript. This cap protects the RNA from degradation and is crucial for the recognition by ribosomes during translation. The unique structure of the cap enhances the efficiency of protein synthesis. It also helps in RNA processing and splicing, reinforcing its significance in ensuring successful translation.
Polyadenylation
Polyadenylation is the addition of a poly(A) tail to the 3' end of the RNA transcript. This modification serves multiple functions, including increasing the stability of the RNA and facilitating its transport out of the nucleus. The poly(A) tail also plays a role in the initiation of translation. It is a beneficial choice because it enhances the lifespan of mRNA in the cytoplasm, allowing for more extensive protein production.
Splicing
Splicing is the process of removing non-coding sequences, known as introns, from the RNA transcript. This is crucial for generating a mature mRNA molecule that only contains the coding sequences, called exons. The splicing process enables a single gene to code for multiple proteins through alternative splicing, showcasing the complexity and flexibility of gene expression. In this light, splicing is fundamental in shaping the final messenger RNA that will guide protein synthesis.
Translation: From RNA to Protein
Translation serves as a critical step in the journey from genetic instructions, held in the structure of DNA, to functional proteins. This process occurs in the cell's cytoplasm, where the messenger RNA, produced during transcription, is decoded into a specific sequence of amino acids. These amino acids then fold into proteins, which are essential for various cellular functions. Understanding translation helps to elucidate how genetic information is expressed and utilized in biological contexts.
Role of Ribosomes
Ribosomes are ribonucleoprotein complexes that play a vital role in translation. They serve as the site where mRNA is translated into protein. Ribosomes consist of two subunits—large and small—each made up of ribosomal RNA and proteins. The small subunit binds to the mRNA strand, while the large subunit facilitates the formation of peptide bonds between adjacent amino acids. This assembly allows ribosomes to read the mRNA codons and align the corresponding transfer RNA molecules, ensuring the correct sequence is assembled. Ribosomes can be found free-floating in the cytoplasm or attached to the endoplasmic reticulum, providing insight into protein synthesis's spatial dynamics and efficiency.
Transfer RNA Function
Transfer RNA (tRNA) plays an essential part in the translation process. Each tRNA molecule carries a specific amino acid and has an anticodon that is complementary to the mRNA codon. When the ribosome encounters an mRNA codon, a corresponding tRNA with the correct anticodon binds to the mRNA. This pairing ensures that the specific amino acid brought by the tRNA is accurately added to the growing polypeptide chain. The efficiency of tRNA in delivering amino acids directly correlates with the accuracy of protein synthesis, emphasizing its significance in maintaining the fidelity of gene expression.
Mechanisms of Translation Initiation
Translation initiation is a multi-step process that kicks off protein synthesis. The small ribosomal subunit assembles around the start codon of the mRNA. This step requires several initiation factors and the input of energy, often in the form of guanosine triphosphate (GTP). Following the assembly of the small subunit, the initiator tRNA, linked to methionine, binds to the start codon. Subsequently, the large ribosomal subunit joins the complex, forming a functional ribosome ready for elongation. This delicate orchestration highlights the phosphorylation and other regulatory mechanisms controlling the transition from mRNA to a functional ribosome.
Elongation and Termination of Protein Synthesis
Once initiation is complete, elongation begins. This phase involves a repetitive cycle where new tRNA molecules enter the ribosome, and amino acids are continuously added to the nascent polypeptide chain. The ribosome moves along the mRNA, and the existing polypeptide chain is transferred to the new amino acid with the aid of peptidyl transferase, an enzymatic function of the ribosome itself.
Termination occurs when the ribosome encounters a stop codon, signaling the end of the protein synthesis. Release factors bind to the stop codon, prompting the ribosome to catalyze a reaction that releases the completed polypeptide chain. This termination stage is crucial for ensuring that proteins are synthesized correctly and that the cell can recycle the ribosome and other components for future translation events.
Translation is a complex but essential process that translates genetic information into functional proteins, serving as a cornerstone of molecular biology.
Translation is a complex but essential process that translates genetic information into functional proteins, serving as a cornerstone of molecular biology.
Understanding translation bridges the gap between genetic sequences and the functional proteins that result, providing insight into cellular processes and the molecule’s role in life.
Post-Translational Modifications
Post-translational modifications represent critical processes that proteins undergo after their synthesis. This section elucidates the significance of these modifications, highlighting how they refine protein function, localization, and overall cellular roles. By adjusting the structural and functional characteristics of proteins, post-translational modifications significantly influence various biochemical pathways in living organisms.
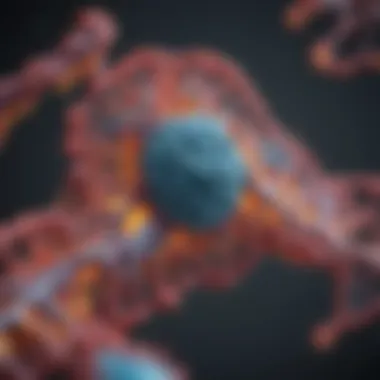
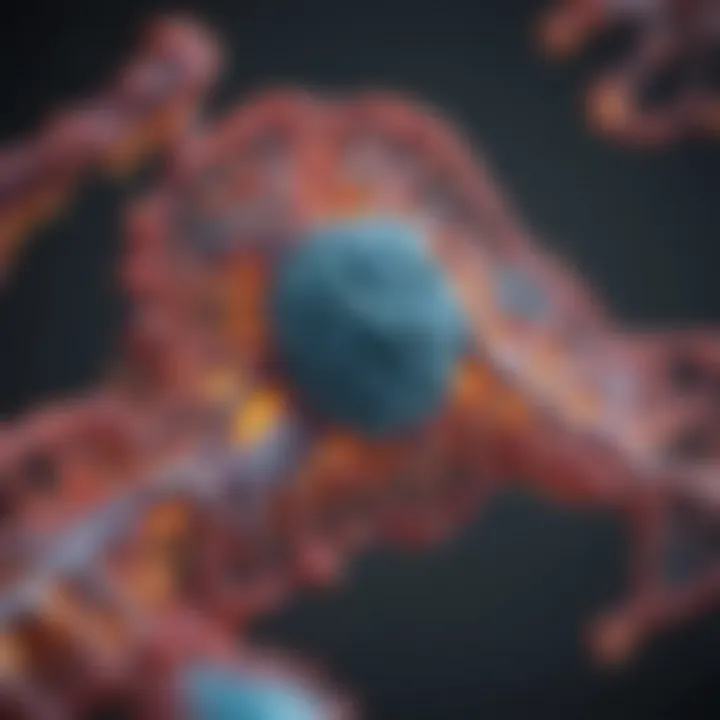
Types of Modifications
Phosphorylation
Phosphorylation is one of the most studied post-translational modifications. It involves the addition of a phosphate group, usually from ATP, to an amino acid residue in a protein. This modification frequently happens on serine, threonine, or tyrosine residues.
The key characteristic of phosphorylation is its reversible nature. Kinases catalyze the addition of phosphate groups, while phosphatases remove them. This cycling between phosphorylated and non-phosphorylated states allows cells to respond dynamically to external signals.
Phosphorylation is a beneficial choice in this context because it can dramatically alter a protein's activity, stability, or localization. For instance, this method is vital in signaling cascades where a signal must be amplified quickly.
However, one potential disadvantage of phosphorylation lies in its regulatory complexity. Incorrect phosphorylation patterns can lead to dysfunctional signaling and may contribute to diseases such as cancer.
Glycosylation
Glycosylation is the addition of sugar moieties to proteins, often occurring in the endoplasmic reticulum and Golgi apparatus. This modification plays a significant role in determining protein structure and function. The type of sugar and how it is linked to the protein can affect a protein's folding, stability, and recognition by other cellular molecules.
The key characteristic of glycosylation is its ability to influence molecular recognition. This can enhance a protein's solubility and protect it from proteolytic degradation. Additionally, glycosylation is critical for cell-cell communication and immune responses.
Glycosylation is a popular modification choice for maintaining cellular homeostasis. However, glycosylation's complexity can lead to variability in protein function, which poses challenges for therapeutic applications where consistent protein behavior is needed.
Ubiquitination
Ubiquitination involves the attachment of ubiquitin, a small protein, to a target protein, usually destined for degradation. This modification acts as a signal for proteasomal degradation, which is essential for regulating protein levels within cells.
A key characteristic of ubiquitination is its role in maintaining cellular protein turnover. It prevents the accumulation of damaged or misfolded proteins, a feature vital for cellular health.
Ubiquitination is beneficial for maintaining protein balance. Nonetheless, the process's complexity can sometimes lead to unintended consequences, such as the degradation of essential proteins and thus causing dysregulation in signaling pathways.
Impact on Protein Function
The impact of post-translational modifications on protein function is extensive. These modifications contribute to the spatial and temporal regulation of proteins. With altered properties, proteins can interact differently with their environment, impacting everything from enzymatic activity to cellular signaling.
"Post-translational modifications serve as fine-tuners of protein function, shaping key biological processes in the cell."
"Post-translational modifications serve as fine-tuners of protein function, shaping key biological processes in the cell."
Through these modifications, proteins can adopt various functional states, enabling responsive and adaptive behavior in fluctuating environments. Understanding these modifications is essential in various fields, including research and medicine, where targeting specific modifications can yield therapeutic benefits.
Regulation of Gene Expression
Regulating gene expression is a fundamental aspect of molecular biology. This process determines how and when genes are turned on or off, influencing protein synthesis and ultimately impacting cellular behaviors. Understanding this regulation is crucial because it plays a significant role in the proper functioning of cells. Without regulation, proteins could be produced in excess or insufficient amounts, leading to various physiological problems.
Gene expression regulation can be categorized into two major processes: transcriptional regulation and translational control. Each of these processes allows for a different level of flexibility and responsiveness to cellular and environmental changes. By finely tuning gene expression, cells can efficiently respond to internal signals and external stimuli.
Transcriptional Regulation
Transcriptional regulation is the first point of control in gene expression. It involves the modulation of RNA polymerase activity, which synthesizes messenger RNA (mRNA) from the DNA template. This regulation is achieved through various mechanisms:
- Transcription Factors: These proteins bind to specific DNA sequences near the gene. They can enhance (activators) or inhibit (repressors) the recruitment of RNA polymerase, affecting the rate of transcription.
- Promoter Regions: The DNA sequence that indicates where transcription begins. Mutations in these areas can have profound effects on gene expression.
- Enhancers and Silencers: Distal regulatory sequences that can increase or decrease transcription levels, respectively. They function through looping to contact the promoter.
The role of epigenetics is also vital in transcriptional regulation. Modifications such as DNA methylation or histone modification can change the accessibility of DNA to transcription machinery without altering the underlying sequence. This allows for dynamic gene expression patterns that can be inherited through cell divisions.
Translational Control
Translational control is the next step after transcription. This process regulates the conversion of mRNA into protein, ensuring that the products of gene expression match the needs of the cell. Mechanisms involved in translational control include:
- mRNA Availability: The stability and life span of mRNA molecules directly impact the amount of protein synthesized. Degradation of mRNA can occur through various pathways, modulating protein output.
- Initiation Factors: Proteins that facilitate the beginning of translation. Their presence can determine the efficiency and rate at which mRNA is translated into protein.
- Ribosomal Regulation: Some ribosomes can selectively translate specific mRNAs based on the cellular context, further refining protein synthesis.
Translational control can be influenced by environmental signals. For instance, stress or nutrient availability can alter the translational landscape, prioritizing the synthesis of proteins essential for survival.
In summary, the regulation of gene expression is a multifaceted process that ensures proteins are synthesized at the right time and in the right amounts. This regulation is essential for cellular function and adaptation, influencing everything from development to responses to environmental changes.
The Role of DNA Mutations
DNA mutations play a crucial part in the genetic landscape of all living organisms. They are deviations from the normal sequence of nucleotides in DNA. Understanding these mutations is imperative in the DNA to protein context as they can significantly impact protein synthesis, structure, and function. In this section, we will explore types of mutations and their repercussions on protein function. This knowledge helps in grasping evolutionary processes, disease mechanisms, and even potential therapies for genetic disorders.
Types of Mutations
Mutations can be categorized into several types based on their origin and effects. Understanding these categories allows researchers to assess their roles in genetic variations and diseases.
- Point Mutations: This is the most common type, where a single nucleotide is altered. It includes substitutions, insertions, and deletions. Each of these can lead to silent mutations, missense mutations, or nonsense mutations.
- Frameshift Mutations: These occur when nucleotides are inserted or deleted from the DNA sequence, causing a shift in the reading frame of the codons. This often results in entirely different and typically nonfunctional proteins.
- Large Scale Mutations: These involve changes that affect larger segments of DNA, such as duplications, inversions, or translocations. They can influence the expression of multiple genes simultaneously.
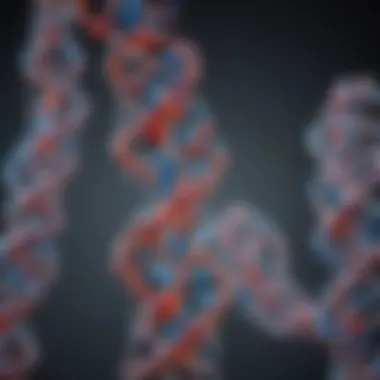
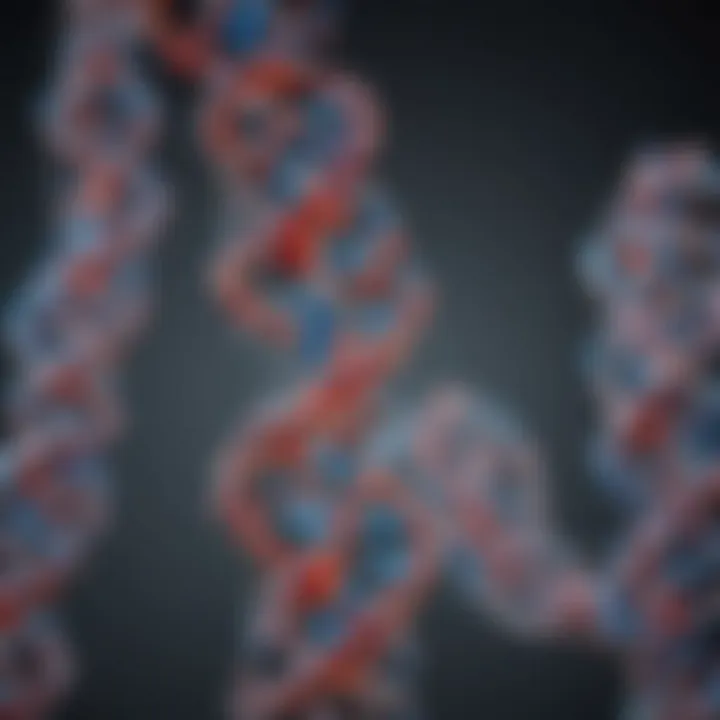
“Mutations are not merely errors; they are the raw material of evolution.”
“Mutations are not merely errors; they are the raw material of evolution.”
Each type affects genetic information differently, leading to a variety of consequences.
Consequences of Mutations on Protein Function
Mutations can affect protein function in multiple ways. Their consequences can be depth and complex, varying greatly depending on the mutation type and its location within the gene.
- Altered Amino Acid Sequence: Point mutations often result in the change of one amino acid in a protein, which can affect folding, stability, or interaction with other molecules. This in turn impacts its functionality.
- Loss of Function: Some mutations lead to complete loss of protein function. Nonsense mutations often introduce premature stop codons, which may produce nonfunctional truncated proteins.
- Gain of Function: In rare instances, mutations can give a protein new activities or enhance existing functions, potentially leading to uncontrolled cellular processes, as seen in some cancers.
- Regulatory Changes: Mutations in non-coding regions can alter regulatory elements of the gene, affecting how genes are expressed and consequently the quantity of protein produced.
In summary, DNA mutations can have profound influences on protein functionality, potentially resulting in a cascade of cellular effects that might lead to diseases or disorders. Understanding these mutations not only helps in the study of molecular biology but also in devising therapeutic strategies.
Applications in Biotechnology
The field of biotechnology has significantly advanced due to our understanding of the DNA to protein process. This knowledge serves as a foundation for numerous applications that impact medicine, agriculture, and industry. Biotechnology harnesses biological systems and organisms to develop technologies and products that improve our quality of life.
One of the key aspects is genetic engineering. This process involves modifying organisms' genomes to produce desired traits. By manipulating DNA, scientists can introduce new characteristics to plants, making them resist pests or tolerate harsh environmental conditions. For instance, genetically modified crops such as Bt corn are engineered to produce their own insecticides. This reduces reliance on chemical pesticides, benefiting both the environment and agricultural productivity.
Another critical application is protein production techniques. As proteins perform most of the functions in a cell, methods to produce them efficiently have gained prominence. Techniques such as recombinant DNA technology allow scientists to produce proteins in microbial systems like E. coli or yeast. These systems can mass-produce therapeutic proteins like insulin, enabling rapid treatment for diabetes patients. Other methods include the use of cell culture systems, which offer a more sophisticated environment for protein production.
"The integration of molecular biology and biotechnology has led to breakthroughs in biopharmaceuticals, diagnostics, and agricultural products."
"The integration of molecular biology and biotechnology has led to breakthroughs in biopharmaceuticals, diagnostics, and agricultural products."
In addition to advancing healthcare, applications in biotechnology raise important considerations. Ethical implications must be addressed, such as the impacts of genetically modified organisms on biodiversity and food security. Regulatory frameworks are essential to ensure that advancements benefit society while minimizing risks.
Biotechnology stands at the intersection of science and application. Understanding the processes from DNA to protein not only paves the way for innovation but also offers solutions to some of the most pressing challenges in health and environmental sustainability. As we continue to explore these applications, researchers are uncovering new methods that redefine possibilities in biotechnology.
Implications for Medicine
The implications of the DNA to protein process in medicine are profound and wide-ranging. Understanding how genetic information translates into functional proteins can lead to significant advancements in diagnostics, treatment strategies, and overall management of diseases. The intricate relationships among genes, their expressed proteins, and the resulting biological functions create a vast landscape for exploration. This section will discuss the relevance of this process in understanding genetic disorders and developing targeted therapies.
Understanding Genetic Disorders
Genetic disorders arise from abnormalities in the DNA sequence that can affect protein synthesis and function. These disorders can be the result of mutations that lead to dysfunctional or absent proteins, disrupting normal cellular processes. Some key points to consider are:
- Types of Genetic Disorders: There are various types of genetic disorders, including single-gene disorders, chromosomal disorders, and multifactorial disorders. Each of these categories encompasses a range of specific conditions, such as cystic fibrosis or Down syndrome.
- Mechanism of Mutation: Changes in the DNA sequence may occur through several mechanisms, including substitution, deletion, or insertion of nucleotides. Understanding these mechanisms is vital for predicting how these mutations influence protein function.
- Impact on Health: The implications of malfunctioning proteins can be severe. Many genetic disorders exhibit symptoms that range from mild to life-threatening, highlighting the necessity of understanding the corresponding DNA bases.
With continuous advancements in genomic research and technology, it is becoming increasingly feasible to identify genetic mutations early, which can have a significant impact on patient outcomes.
Targeted Therapies and Personalized Medicine
Targeted therapies represent a promising avenue in the treatment of various medical conditions informed by an understanding of the DNA to protein process. Here are some aspects to consider:
- Mechanism-Based Approaches: Targeted therapies focus on specific molecules involved in disease processes. For instance, many cancer treatments target specific proteins that stem from mutated genes. By understanding how these mutations affect the corresponding protein, clinicians can devise more effective treatment protocols.
- Personalized Medicine: The understanding of individual genetic profiles enables healthcare providers to tailor treatments based on a patient's unique genetic makeup. This approach increases the likelihood of therapeutic success and minimizes adverse effects, as therapies can be optimized for each patient's specific genetic context.
- Future Directions: With the rise of CRISPR technology and gene editing, the potential to correct genetic defects at the DNA level offers hope for revolutionary treatments for genetic disorders. This can lead to permanent solutions rather than temporary alleviation of symptoms.
"The shift towards precision medicine and targeted therapies highlights the importance of understanding the molecular basis of disease, making the journey from genes to proteins an essential area of study in modern medicine."
"The shift towards precision medicine and targeted therapies highlights the importance of understanding the molecular basis of disease, making the journey from genes to proteins an essential area of study in modern medicine."
In summary, the relationship between the DNA to protein process and medicine underscores the critical role that molecular biology plays in understanding diseases and developing innovative therapies that can transform patient care.
Epilogue
The conclusion of this article encapsulates the significant elements surrounding the DNA to protein process. This process is fundamental to understanding how genetic information is transcribed and translated into functional proteins, which play indispensable roles in biological systems. The importance of this topic extends beyond basic biology.
Summary of the DNA to Protein Process
In summary, the DNA to protein process describes the intricate mechanisms that cells utilize to convert genetic information into proteins. This journey begins with the transcription of DNA into messenger RNA (mRNA). This mRNA is then translated by ribosomes into polypeptide chains, which later fold into functional proteins. The specific steps include:
- Transcription: DNA is unwound and a complementary RNA strand is synthesized.
- Translation: Ribosomes facilitate the decoding of mRNA into amino acids.
- Post-Translational Modifications: Proteins undergo various modifications that can affect their functionality.
Each of these stages is regulated carefully, ensuring that proteins are synthesized according to the cell’s needs. Disruptions in any phase can lead to cellular dysfunction and diseases, emphasizing the relevance of understanding this topic.
Future Directions in Research
Looking ahead, several avenues of research seem promising for the DNA to protein field. Potential future directions include:
- Gene Editing Technologies: Advancements in CRISPR and related technologies offer insights into targeted modifications of genes, enhancing our ability to correct mutations that cause genetic disorders.
- Protein Folding Studies: Understanding how proteins attain their functional shapes could lead to breakthroughs in treating diseases associated with misfolded proteins.
- Synthetic Biology: As synthetic biology advances, the potential to create novel proteins with specific functionalities presents exciting opportunities for medicine and industry.
The interplay between DNA, RNA, and proteins remains a cornerstone of molecular biology. Continuous research will deepen our understanding of life at a molecular level and its implications for health and disease.
The interplay between DNA, RNA, and proteins remains a cornerstone of molecular biology. Continuous research will deepen our understanding of life at a molecular level and its implications for health and disease.
In summary, comprehending the DNA to protein process not only sheds light on the cellular function but also helps pave the way for innovative medical therapies and biotechnological applications.