Understanding Protein Synthesis from DNA
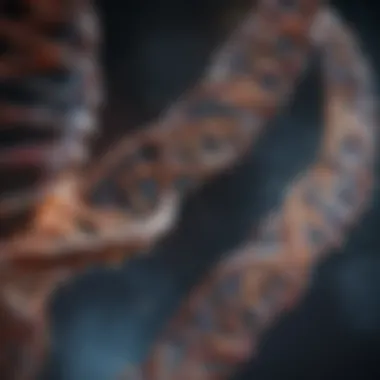
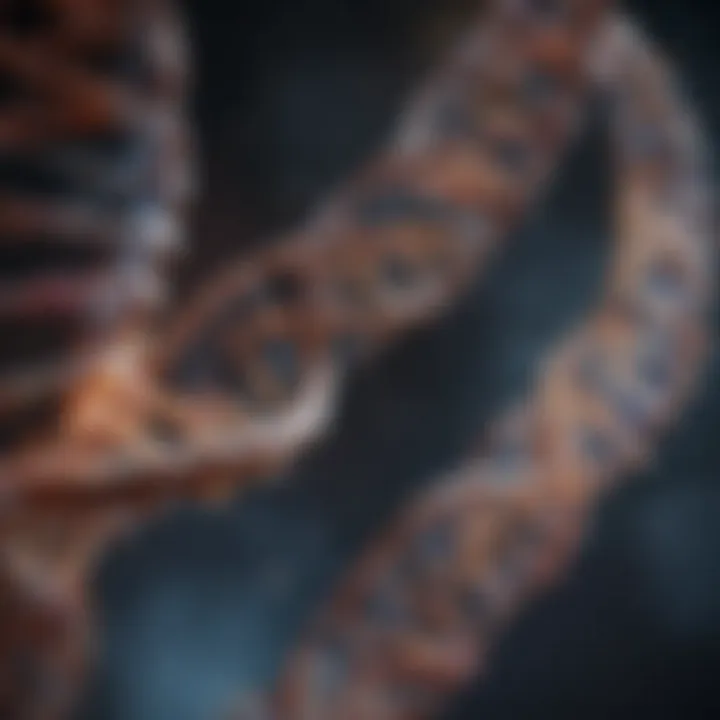
Intro
The journey from DNA to proteins is not just a biological process; it is a symphony of molecular interactions that fuels life itself. Encompassing a series of meticulously orchestrated steps, this process governs how genetic information is converted into functional proteins, which perform a multitude of roles in organisms. Understanding this transition from the blueprint of life—DNA—to the crucial workhorses of the cell—proteins—is fundamental for students, researchers, educators, and professionals alike.
Key Concepts
Definition of the Main Idea
At its core, the transformation from DNA to proteins illustrates the central dogma of molecular biology, emphasizing a one-way street from DNA to RNA, and then to proteins. This means that genetic codes held in DNA are first transcribed into messenger RNA (mRNA) and later translated into the language of proteins. Each amino acid, the building blocks of proteins, corresponds to a three-nucleotide sequence in the mRNA, known as a codon. The precision of this translation is vital for the proper functioning of cellular processes.
Overview of Scientific Principles
The molecular journey begins in the nucleus, where DNA resides. Here, enzymes like RNA polymerase play a pivotal role in the transcription phase, unzipping the DNA strands and synthesizing mRNA from its template. Once synthesized, this mRNA exits the nucleus through nuclear pores, entering the cytoplasm where translation occurs.
During translation, ribosomes create proteins by reading the mRNA sequence in sets of three nucleotides (codons). Transfer RNA (tRNA) molecules ferry the appropriate amino acids to the ribosome, ensuring that the protein is assembled correctly according to the mRNA instructions.
The regulation of gene expression is equally important. Various factors, such as transcription factors, help dictate when and how much of a protein is made, impacting everything from cellular function to an organism’s development.
Current Research Trends
Recent Studies and Findings
Recent advancements in genetic technologies, such as CRISPR and RNA sequencing, have provided deeper insights into this molecular journey. Researchers are now exploring how small modifications in transcription and translation can impact protein functions and lead to diseases. Moreover, studies have shown the influence of non-coding RNAs in regulating protein synthesis, an area that remains active in research.
Significant Breakthroughs in the Field
One noteworthy breakthrough has been the mapping of ribosome structures, which allows scientists to understand how proteins are translated with high fidelity. This knowledge opens avenues for developing therapies targeting ribosomal function in diseases like cancer.
"Understanding protein synthesis is not just an academic pursuit; it lays the groundwork for innovative treatments and biotechnology applications that can alter the course of diseases."
"Understanding protein synthesis is not just an academic pursuit; it lays the groundwork for innovative treatments and biotechnology applications that can alter the course of diseases."
In summary, exploring the intricate processes that take place from DNA to proteins reveals the complex network of interactions that sustain life, highlighting the relevance of rigorous research in both genetic engineering and disease treatment. The implications of understanding these processes are profound, emphasizing the need for continued investigation into this fascinating molecular journey.
Prologue to Molecular Biology
Molecular biology serves as the cornerstone of understanding the complex interplay between genetic information and biological function. It delves into how life is orchestrated at a molecular level, illuminating the crucial processes that enable living organisms to maintain themselves, reproduce, and evolve. Exploring molecular biology not only serves an academic purpose but also has profound implications in various fields such as genetics, medicine, and biotechnology.
With DNA as the fundamental blueprint of life, comprehending its structure and operational mechanisms is paramount. This foundation paves the way for appreciating how genes are expressed and regulated within cells, a critical aspect of both health and disease. The importance of this field transcends mere theoretical knowledge; it catalyzes practical applications, driving innovations in gene therapy, personalized medicine, and agricultural biotechnology.
One must also acknowledge the evolving landscape of molecular biology. Advances in technology, particularly in genomics and proteomics, empower researchers to delve deeper into the complexities of genetic material and its transformations. As a result, the study of molecular biology provides critical knowledge that enriches our understanding of life itself and facilitates tackling global challenges, including infectious diseases and genetic disorders.
"Molecular biology is like a well-tuned orchestra, where each instrument plays a unique role in creating the symphony of life."
"Molecular biology is like a well-tuned orchestra, where each instrument plays a unique role in creating the symphony of life."
Understanding DNA Structure
The structure of DNA, or deoxyribonucleic acid, is often characterized as a double helix, resembling a twisted ladder. This architecture is not just a random configuration; it is crucial to DNA's function. Composed of nucleotide building blocks, each consisting of a sugar, phosphate group, and a nitrogenous base, DNA's stability and flexibility arise from this structure. The tight coiling of the double helix allows for efficient storage of genetic information within the confines of a cell's nucleus.
The four types of nitrogenous bases—adenine, thymine, cytosine, and guanine—form specific pairs (A-T and C-G) that play a critical role in the fidelity of genetic information. Understanding this pairing mechanism is essential because it ensures that during replication, the genetic code is faithfully transmitted to daughter cells. Moreover, this specificity sees its significance in various biotechnological applications, such as PCR (Polymerase Chain Reaction) and DNA sequencing methods.
In addition, the sequence of these bases is what encodes the instructions needed for constructing proteins, the building blocks of life. Thus, a profound understanding of DNA structure is the first step in grasping how the molecular journey from DNA to proteins unfolds.
Histones and Chromatin: The Packaging of DNA
DNA does not exist freely in the nucleus; instead, it is intricately packaged into a structure known as chromatin, which ensures that the long strands of DNA fit inside the tiny nucleus of a cell. This packaging is primarily facilitated by proteins called histones.
Histones act like spools around which DNA winds, forming nucleosomes. This process of winding and unwinding is crucial for regulating access to genetic material. When genes need to be expressed, chromatin becomes more open, allowing the transcription machinery to interact with the DNA. Conversely, when certain genes need to be silenced, the chromatin adopts a more compact structure, effectively restricting access to that DNA.
- Understanding how chromatin structure influences gene expression leads to valuable insights into both normal cellular function and pathological states, such as cancer. This fine-tuning of accessibility is not static; it can change in response to various signals, effectively enabling the cell to adapt to its environment.
In summary, both histones and chromatin play pivotal roles in ensuring that DNA is not only stored efficiently but also regulated at critical junctures, marking important nodes in the molecular journey from DNA to proteins.
The Central Dogma of Molecular Biology
The central dogma of molecular biology serves as the compass for understanding how information encoded in DNA translates into functional proteins. This pivotal framework underpins the narrative of life itself, where nucleic acid sequences dictate cellular behavior, warranting a closer inspection of its multifaceted mechanisms. Within this journey, pathways like transcription and translation emerge as vital processes that not only elucidate molecular processes but also highlight the significance of gene regulation. In this article, we will delve deeper into these processes, illustrating their relevance and implications.
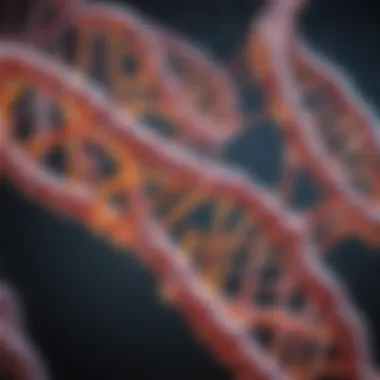
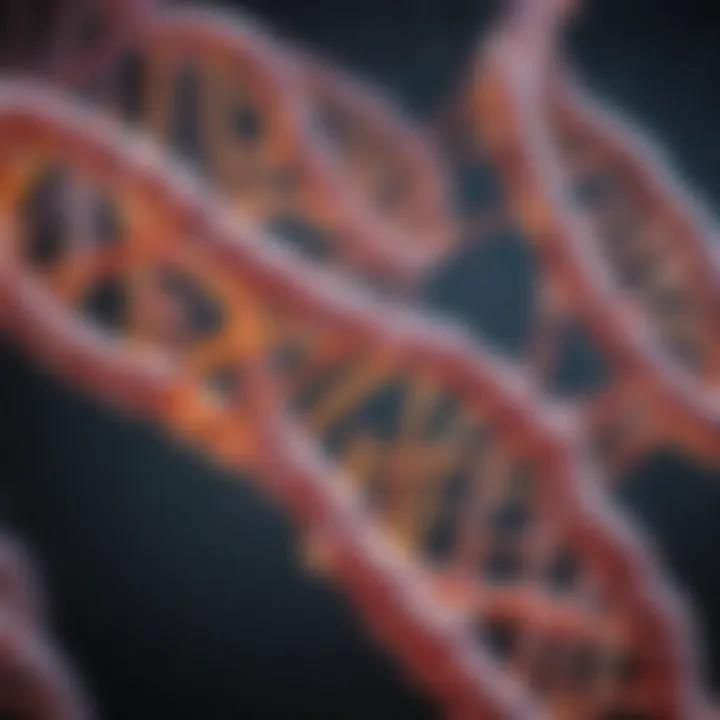
Transcription: From DNA to mRNA
Initiation of Transcription
Initiation of transcription marks the crucial first step in the synthesis of messenger RNA (mRNA) from a DNA template. This phase is characterized by the assembly of various proteins and enzymes at specific DNA regions known as promoters. A key feature of initiation is the role of RNA polymerase, which recognizes and binds to the promoter site. This unique property allows it to kick-start the transcription process, making it an indispensable factor in gene expression. The beauty of this process lies in its ability to precisely regulate when and where genes are activated, thus contributing significantly to cellular function.
Ordinarily, a lack of efficient promotion can stall transcription, leading to lesser protein production and affecting overall cellular health.
Elongation and Termination
Elongation and termination follow the initiation phase, playing a pivotal role in extending the nascent mRNA chain and concluding its synthesis. During elongation, RNA polymerase unwinds the DNA and adds RNA nucleotides complementary to the DNA template. One of the notable characteristics of this phase is the rapid production of mRNA, allowing the cell to respond swiftly to its environment, thereby emphasizing its metabolic adaptability.
Termination brings about the end of the transcription process when RNA polymerase recognizes specific sequences that signal, effectively ceasing synthesis. This duality of elongation and termination, though seemingly straightforward, showcases the intricate mechanisms ensuring that only the right amount of mRNA is produced at any given time.
RNA Processing
M RNA processing is essential in refining the initially produced mRNA, ensuring it is fully functional. This process typically involves the addition of a 5' cap and a poly-A tail, along with the splicing of non-coding regions known as introns. The unique feature of this stage is that it allows for a single gene to be expressed in various ways through alternative splicing, enabling the generation of multiple protein variants.
However, mRNA processing comes with its own complexity. It requires teamwork among several molecular players, like spliceosomes, which can occasionally lead to errors. Such mishaps could result in non-functional proteins or even diseases, underscoring the delicate balance maintained in gene expression.
Translation: From mRNA to Protein
The Role of Ribosomes
Ribosomes act as the manufacturing plants of the cell, translating the mRNA blueprint into a corresponding protein. Each ribosome comprises rRNA and proteins, working together seamlessly to decode mRNA into its respective amino acids. A significant aspect of ribosomes is their ability to initiate translation at the start codon, guiding tRNA to bring the appropriate amino acids.
One key characteristic of ribosomes is their efficiency in synthesizing proteins rapidly, sometimes producing several polypeptides simultaneously. The sheer volume of protein synthesis reflected in this process highlights its fundamental importance in maintaining cell integrity and function.
Transfer RNA (tRNA) Functionality
Transfer RNA serves as the key communicator between the mRNA sequence and the amino acids to be assembled into a protein. Each tRNA molecule has an anticodon that pairs with its corresponding codon on the mRNA, ensuring that the correct amino acid is brought to the growing polypeptide chain.
The beauty of tRNA is its specificity. Each tRNA recognizes only one amino acid, tailoring the protein synthesis process with accuracy. Nonetheless, the dependency on a multitude of tRNA molecules can present challenges if any undergo mutations or depletion, which may lead to incorrect protein assembly.
Peptide Bond Formation
Peptide bond formation is the actual mechanism that links amino acids together, resulting in a polypeptide chain. This process occurs within the ribosome and relies on the enzymatic activity of rRNA.
One important aspect of peptide bonds is the stability it provides to proteins once formed, ensuring durability while allowing for flexibility in protein folding. However, the challenges arise when disruptions occur in the bond formation mechanisms, this can lead to misfolded proteins and associated diseases.
"Understanding the central dogma journeys not just through molecular biology, but also across the very essence of living organisms."
"Understanding the central dogma journeys not just through molecular biology, but also across the very essence of living organisms."
In summary, the central dogma lays the foundational narrative for how genetic information flows from DNA into functional proteins. Each step, from transcription to translation, is marred by potential pitfalls but also arrayed with opportunities for cellular command and adaptability.
The exploration of these mechanisms not only enhances our grasp of life’s molecular underpinnings but also sets the stage for advancements in fields ranging from medicine to genetic research.
The Genetic Code and Its Importance
The genetic code serves as the fundamental framework upon which life is built. It dictates the relationship between nucleic acids, which we know as DNA, and proteins, the building blocks of cellular structures and functions. Understanding the genetic code goes beyond mere academic exercise; it offers profound insights into the functioning of life itself and has led to advancements in biotechnology, medicine, and evolutionary biology.
The significance of the genetic code can be highlighted through several key aspects:
- Universal Language: The code is remarkably universal across various forms of life, showcasing the shared ancestry of organisms. This means that a codon in a human can code for the same amino acid in a fruit fly without a hitch.
- Conservation of Function: Because of the code's consistency, scientists can predict protein functions and behavior across species. This has tremendous applications in biotechnology and medicine, where understanding disease mechanisms in one organism can inform treatment strategies in another.
- Connections to Evolution: Mutations play a crucial role in evolutionary changes. The genetic code helps in tracing back these mutations to understand how species adapt over time.
"Understanding the genetic code is like possessing a map to a hidden treasure, revealing the intricate journey from genes to physical traits."
"Understanding the genetic code is like possessing a map to a hidden treasure, revealing the intricate journey from genes to physical traits."
Thus, the genetic code encapsulates the essence of biological information transfer. Appreciating its complexities and implications paves the path to deeper biological understanding and innovation.
Understanding Codons
At the heart of the genetic code are codons, sequences of three nucleotides in DNA or RNA that correspond to specific amino acids. This triplet coding system is vital; it determines which amino acids are added during protein synthesis. The reason for using groups of three is linked to the need for sufficient variability. With only four nucleotides (adenine, guanine, cytosine, and uracil in RNA), a combination of three allows for 64 possible codons, which is more than enough to code for the 20 standard amino acids.
Each codon sends a critical message to the ribosome, the cellular machinery responsible for building proteins. For example:
- The codon AUG signals the start of protein synthesis, known as the start codon.
- Other codons specifically code for amino acids like UUU (phenylalanine) or GGC (glycine).
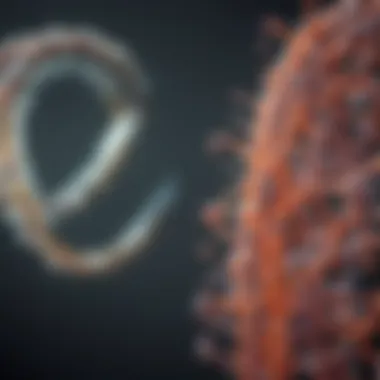
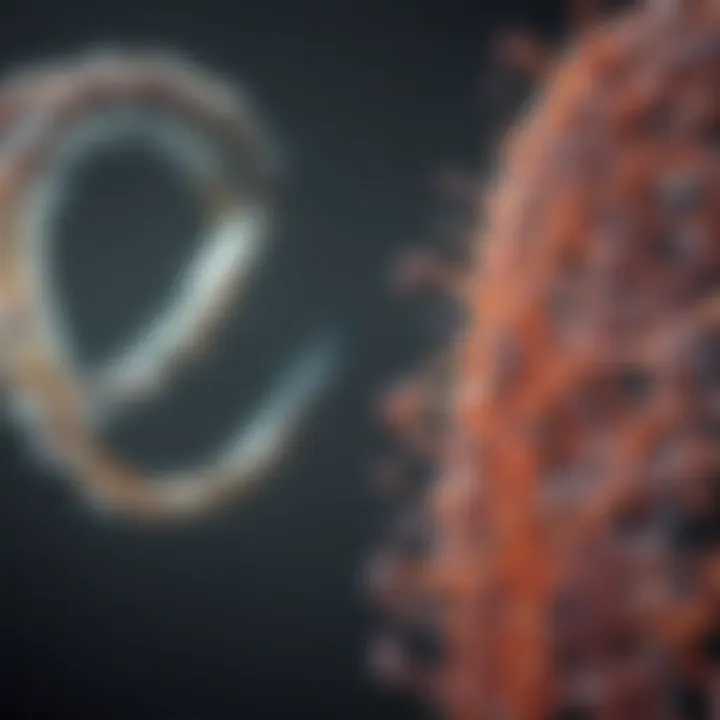
Additionally, codons do not operate in isolation. They exist in a larger context within mRNA, with regulatory sequences influencing how effectively the ribosome translates them into proteins. Moreover, synonymous codons—those that code for the same amino acid—can impact protein folding and functionality in more subtle ways.
Start and Stop Codons
The beginning and end of protein synthesis are marked by start and stop codons, which play a crucial role in ensuring that proteins are created accurately and efficiently.
- Start Codons: The most well-known start codon is AUG. It not only signals the beginning of translation but also codes for methionine, the first amino acid in the majority of proteins. The presence of AUG is paramount because it sets the reading frame; if it were to shift due to an error, subsequent codons would lead to incorrect amino acid incorporation, potentially leading to dysfunctional proteins.
- Stop Codons: Unlike start codons, stop codons do not code for any amino acids. They – UAA, UAG, and UGA – signal the termination of protein synthesis. When a ribosome encounters a stop codon, it releases the completed polypeptide chain to undergo folding and eventual functionality in the cell. This system ensures that proteins are synthesized with the correct length and composition; a missed stop codon can result in extended proteins and disrupt cellular function.
In summary, understanding codons, particularly start and stop codons, is vital for grasping how genes translate into functional proteins. Whether in a classroom or a research lab, the mechanics of these molecular signals can catalyze numerous discussions on genetic interactions and applications in synthetic biology.
Regulation of Gene Expression
The regulation of gene expression fundamentally governs how cellular information is utilized and transformed into functional proteins. This dynamic process is paramount since it determines not just when genes are turned on or off but also how much product is produced. Without this regulatory framework, cells would be overwhelmed with unwanted proteins, leading to inefficiencies and potential harm. Understanding gene expression regulation enhances our grasp of biology and its applications in fields like medicine and biotechnology.
Operons in Prokaryotes
Operons serve as a classic example of gene regulation in prokaryotes. In essence, an operon is a cluster of genes that are transcribed together under the control of a single promoter. This means that they can be turned on or off collectively. One of the most studied operons is the lac operon found in Escherichia coli, which is responsible for the metabolism of lactose. When lactose is present, it binds to a repressor protein, modifying its shape and preventing it from blocking the promoter. This allows RNA polymerase to access the genes in the operon, resulting in their transcription.
Some key characteristics of operons include their efficiency in resource utilization and the ability to rapidly respond to environmental changes. However, they also come with inherent limitations. For instance, if a mutation affects the promoter, it can lead to the entire pathway being affected, thereby disrupting cellular functions. Overall, operons exemplify how simple organisms manage their genetic resources efficiently.
Eukaryotic Gene Regulation
Transcription Factors
Transcription factors are pivotal in the regulation of gene expression in eukaryotic cells. These are proteins that bind to specific sequences of DNA near the gene they regulate, either enhancing or silencing transcription. The versatility of transcription factors makes them a topic of great interest, given their role in various cellular processes including development, differentiation, and response to environmental stimuli.
A notable feature of transcription factors is their ability to interact with various other proteins, forming complexes that can either open up or compact chromatin structure. This is a significant characteristic because chromatin structure plays a direct role in whether a gene can be accessed and transcribed. For instance, the presence of certain transcription factors can lead to an active chromatin state, exposing genes for transcription.
However, one must consider that while transcription factors allow for precise regulation, misregulation can lead to severe consequences, such as cancer. The flexibility of transcription factors is thus a double-edged sword.
Epigenetic Modifications
Epigenetic modifications add another layer of complexity to gene regulation. These are chemical changes to DNA or histone proteins that affect how genes are expressed without altering the underlying genetic sequence. This includes processes like DNA methylation, where methyl groups are added to DNA, typically silencing genes. They contribute significantly to cellular diversity and specialization in multicellular organisms.
The crux of epigenetics is that it provides a mechanism for cells to adapt and respond to their environment. A key characteristic is its ability to be transient – modifications can be reversed, allowing cells to reprogram themselves in response to changes. However, the very nature of epigenetic changes can also lead to problems if the mechanisms fail or are improperly regulated, which can result in developmental disorders or cancer.
In summary, the regulation of gene expression is a multifaceted concept that underpins fundamental biological processes. By investigating operons in prokaryotes and the roles of transcription factors and epigenetic modifications in eukaryotes, we gain crucial insights into how life adapts, evolves, and responds to the environment.
Mutations and Their Effects
Understanding mutations is crucial. They act like tiny fraying threads in the fine tapestry that is our genetic code. Mutations can arise from various sources: environmental factors, replication errors during cell division, or even random chance. Each mutation presents a unique story. While some can lay the groundwork for diseases or conditions, others might just be neutral, sitting quietly in our DNA without causing trouble. In the realm of biotechnology and genetics research, recognizing mutations allows scientists to pair outcomes with origins, and this ability enhances both studies in healthcare and agriculture.
The implications of mutations are broad and far-reaching. After all, mutations can lead to the evolution of species, adaptation to environmental pressures, and a host of genetic diversity. The ability to adapt is often what ensures the survival of species over eons. However, not all mutations are beneficial—some can lead to genetic diseases, cancers, or other health-related problems.
The type of mutations primarily fall into two categories, which we’ll dive into below.
Types of Mutations
Mutations can be categorized in various ways, but two primary types come to light in our discussion: point mutations and frame shift mutations. Understanding these types is like identifying the basic building blocks of genetic changes.
- Point Mutations: This is when a single nucleotide is altered in the DNA sequence. It’s akin to changing a word in a sentence – sometimes it alters the meaning, sometimes it doesn’t.
- Frame Shift Mutations: On the other hand, frame shift mutations occur when nucleotides are added or deleted from the sequence. This not only changes one word but can shift the entire paragraph, often leading to significant changes in the resulting protein.
Consequences of Mutations
The aftermath of mutations can either be profound or negligible. While some are silent, others can set off a chain of reactions that affect cellular health.
Point Mutations
Point mutations hold a unique place in the genetic narrative because they can lead to significant changes but often might have subtle effects. A point mutation may replace one amino acid for another in a protein sequence. For instance, the sickle cell mutation is a well-known example where just a single nucleotide change creates a completely different behavior in hemoglobin molecules.
- Key Characteristics: The most telling feature of point mutations is that they are relatively straightforward. They can cause missense mutations, where the amino acid changes, or silent mutations, where the overall protein remains unchanged despite the DNA switch.
- Advantages/Disadvantages: The beauty of point mutations is their varied impact. While they can lead to beneficial traits, such as increased resistance to certain diseases, they also carry risks, including genetic disorders.
Frame Shift Mutations
In contrast, frame shift mutations are typically seen as more damaging. These mutations create a new sequence of codons, potentially altering the entire amino acid sequence downstream from the change. This is like moving the goalposts mid-game; suddenly, the rules of protein synthesis shift.
- Key Characteristics: Frame shifts often produce nonfunctional proteins, and the earlier in the sequence they occur, the more detrimental the effects. For this reason, they are often a point of concern in genetic studies.
- Advantages/Disadvantages: While frame shift mutations are less likely to make a beneficial impact due to how dramatically they change the genetic message, they can sometimes lead to novel protein functions. Such changes may enhance adaptability in changing environments, underscoring nature's unpredictable dynamics.
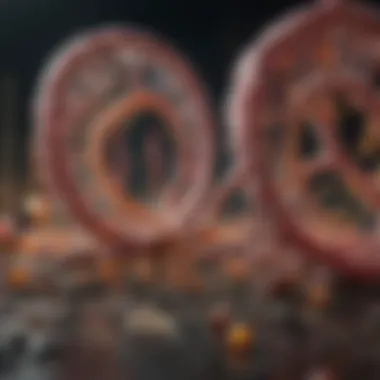
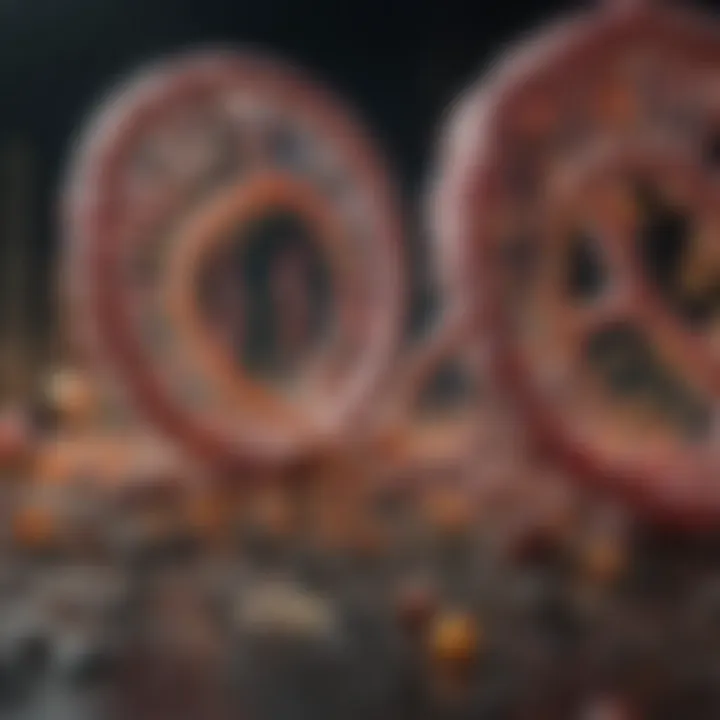
In summary, the role of mutations in genetics is complex and layered. They are the raw material of evolution, mysteries waiting to be unraveled. As we continue our exploration of the molecular journey from DNA to proteins, understanding these mutational changes provides a deeper insight into life itself, the variance, and the constant dance between order and disorder.
In summary, the role of mutations in genetics is complex and layered. They are the raw material of evolution, mysteries waiting to be unraveled. As we continue our exploration of the molecular journey from DNA to proteins, understanding these mutational changes provides a deeper insight into life itself, the variance, and the constant dance between order and disorder.
Biotechnology and Its Applications
Biotechnology has taken center stage in the contemporary narrative of science and health. Its potential is profound, reshaping how we view genetics and molecular biology.
In discussing biotechnology, the focus is two-fold. First, it’s essential to highlight genetic engineering as a frontier of biotech advancements. Secondly, applications in medicine and research carve a path that can significantly affect lives.
Imagine a world where diseases can be tackled at their genetic roots. This is the crux of biotechnology, enabling targeted interventions that were once thought impossible.
As we embark on this exploration, it’s clear that biotech offers myriad benefits, such as:
- Enhanced crop yield through genetically modified organisms (GMOs)
- Treatments for genetic disorders via gene therapy
- Innovative therapies for complex diseases like cancer
However, these advancements necessitate careful consideration of ethical implications and potential long-term consequences, ensuring that society navigates this intricate terrain responsibly.
Genetic Engineering Techniques
CRISPR-Cas9
CRISPR-Cas9 stands out as a revolutionary tool in genetic engineering, enabling precise editing of DNA sequences. What truly sets it apart is its ability to target specific genes with unprecedented accuracy, making modifications that would have taken years or may have been impossible before.
This technique has gained popularity due its robust simplicity and efficiency—scientists are able to deliver results faster and often at a lower cost than traditional methods.
Its key characteristic is the guided RNA, which directs the CRISPR system to the precise location in the genome for cutting. The advantage of this specific targeting means fewer off-target effects, ideally resulting in safer interventions.
However, concerns linger regarding unintended genomic alterations, which can lead to debates about the ethics of editing human germlines.
Recombinant DNA Technology
Recombinant DNA technology involves combining genetic material from different sources. This method has been pivotal in creating organisms, cells, or molecules with altered properties. In terms of biotechnological applications, this technique plays an essential role in gene cloning, where desired traits can be inserted into host organisms.
The key feature here is its versatility—one can use it to produce proteins, hormones, or vaccines. Recombinant DNA technology remains beneficial mainly due to its wide-ranging applications from producing insulin to designing plant varieties resistant to pests.
However, as with CRISPR, there are challenges, such as potential ecological impacts and concerns over the safety and stability of genetically modified organisms.
Applications in Medicine and Research
Biotechnology’s contributions extend deeply into medicine, revolutionizing how we treat ailments. Applications range from the development of biopharmaceuticals and regenerative medicine to personalized treatments based on individual genetic profiles.
In research, biotech facilitates breakthroughs in understanding diseases at the molecular level, leading to innovative diagnostics. For instance, monoclonal antibodies developed through biotechnological methods are now essential tools in identifying and treating different forms of cancer.
Overall, the infusion of biotechnology into medicine and research not only enhances therapeutic options but also reshapes the future landscape of health science. As we delve into these applications, it must be acknowledged that this is only the beginning; the full potential of biotechnology has yet to be fully realized.
Closure: The Role of DNA in Living Organisms
The significance of the role of DNA in living organisms cannot be overstated. Throughout this article, we have peeled back the layers to reveal the intricate mechanisms that drive life itself. DNA serves as the blueprint for all biological functions. It dictates the structure and function of proteins, which are essential for almost every process within a cell. From enzyme activity to cellular structure, the influence of DNA is omnipresent and foundational.
In essence, DNA does not work in isolation. It interacts dynamically with various cellular machinery, which includes transcription factors, RNA polymerase, and ribosomes. This orchestration is what allows genetic messages to be accurately conveyed and translated into functional proteins. As elucidated earlier, regulation is vital; the operons in prokaryotes and the complex eukaryotic regulatory mechanisms ensure that the right genes are expressed at the right time.
Moreover, understanding DNA extends well beyond its role in protein synthesis. It encompasses genetics, biodiversity, and evolutionary biology. The hereditary material of an organism influences traits, behaviors, and adaptability - elements that are crucial in the study of evolution.
In summary, the study of DNA has far-reaching implications. It informs not just areas of molecular biology but fields like medicine, agriculture, and biotechnology. The insights gained from research on DNA contribute to numerous advancements, such as gene therapy and advancements in personalized medicine. Perhaps most importantly, the more we uncover about DNA, the clearer it becomes: this molecule is at the heart of life.
The Fundamental Nature of Genetic Material
At its core, DNA can be considered the foundational element of genetic material. This double-helical structure, made up of nucleotides, encodes the information necessary for the development and function of all living organisms. It’s fascinating how just four nucleotides can create infinite variations of genetic information.
DNA encompasses genetic instructions that define the traits of an organism. Each sequence of nucleotides corresponds to particular proteins, which play critical roles in the body. For instance, the protein hemoglobin, which carries oxygen in the blood, is produced based on the instructions encoded in DNA.
Moreover, the stability of DNA is part of what makes it an effective repository for genetics. While mutations can occur, leading to variations, the overall structure remains remarkably intact across generations. This ensures continuity of genetic information, facilitating both evolution and adaptation through natural selection.
- Robustness: The DNA's ability to repair itself and maintain integrity is vital for survival.
- Variability: Mutations, while sometimes harmful, can lead to beneficial adaptations.
- Transmission: DNA is passed down through generations, making it essential for inheritance.
As we look toward the future, we must appreciate that while we have grasped much about DNA's function, its deeper meanings and implications for biology continue to unfold.
Future Directions in Genetic Research
The frontiers of genetic research are vast and teeming with potential. As technology advances, the exploration of DNA and its functions become more intricate. A few emerging directions are worth noting:
- CRISPR and Gene Editing: This revolutionary technology allows scientists to make precise alterations to DNA, which could lead to cures for genetic diseases.
- Epigenetics: Beyond the genetic code itself, understanding how environmental factors influence gene expression broadens our insight into heredity.
- Synthetic Biology: The ability to design and construct new biological parts offers exciting applications in medicine, materials science, and more.
- Genomic Sequencing: As sequencing technology continues to improve, we can expect more comprehensive insights into genetic diversity and its implications for populations.
- Personalized medicine could become a reality if targeted therapies are developed.
- This could have implications for mental health and chronic diseases.
- Imagine programming cells to produce pharmaceuticals on demand.
- This may revolutionize our understanding of evolution and population dynamics.