Understanding DNA: Structure, Function, and Significance
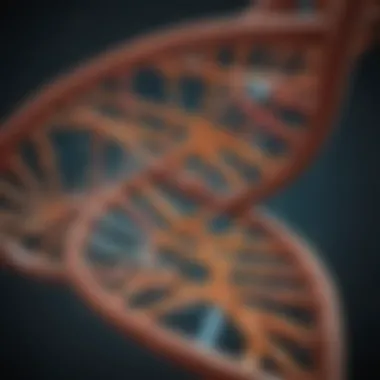
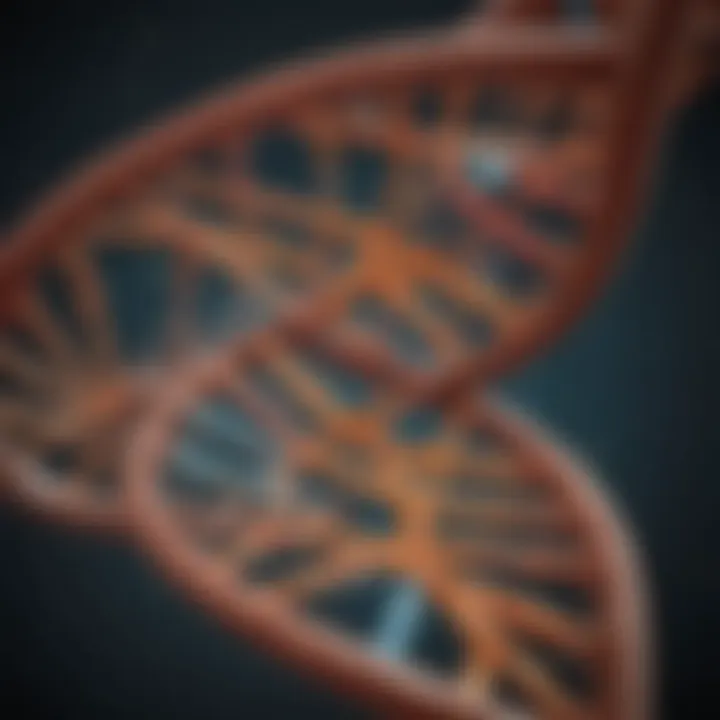
Intro
Deoxyribonucleic acid, commonly known as DNA, is a pivotal molecule that serves as the blueprint for all living organisms. Its complex double helix structure and the unique functions it performs make it a fundamental element of life. By unraveling its intricacies, scientists have ventured into a realm that not only enhances the understanding of biology but also paves the way for advances in medicine, biotechnology, and forensics.
In this article, we shall embark on a journey into the world of DNA, exploring its structural elements and functions in detail. This exploration is not merely an academic exercise but a necessary inquiry into how genetic information is stored, expressed, and transmitted.
Key Concepts
Definition of the Main Idea
At its core, DNA is a molecule consisting of two strands that coil around each other, forming a structure often likened to a twisted ladder. The rungs of this ladder are made up of nucleotide bases: adenine (A), thymine (T), cytosine (C), and guanine (G). These bases pair specifically—A with T and C with G—creating a sequence that encodes genetic information.
However, DNA's significance extends beyond mere structure. It acts as the directive code for building proteins, which are vital for countless biological processes. The interplay between DNA, RNA, and proteins forms a complex web that is essential for life.
Overview of Scientific Principles
To grasp how DNA functions, one must understand some key scientific principles:
- Replication: The process by which DNA makes a copy of itself, ensuring genetic continuity during cell division.
- Transcription: This is where the information in a DNA sequence is converted into an RNA molecule, which serves as a template for protein synthesis.
- Translation: The actual synthesis of proteins based on the RNA template, occurring in ribosomes of the cell.
Understanding these principles illuminates the mechanisms behind hereditary traits and the myriad of functions that proteins perform within the organism.
Current Research Trends
Recent Studies and Findings
In recent times, research on DNA has surged, particularly in the realms of genetic editing and disease prevention. Techniques such as CRISPR-Cas9 have reshaped the landscape of genetic engineering, enabling precise modifications to DNA sequences. Ongoing studies focus on the ethical implications and potential applications of this technology, particularly in treating genetic disorders and enhancing agricultural productivity.
Significant Breakthroughs in the Field
The unveiling of the complete human genome in 2003 marked a significant milestone in genetics. This achievement paved the way for personalized medicine by allowing researchers to identify genetic predispositions to diseases. Additionally, advancements in sequencing technologies have dramatically reduced the cost and time required to decode DNA, making large-scale genomic studies more feasible than ever before.
"With the rise of genome-editing technologies, our understanding of DNA has transitioned from a theoretical framework to a practical toolkit capable of altering life itself."
"With the rise of genome-editing technologies, our understanding of DNA has transitioned from a theoretical framework to a practical toolkit capable of altering life itself."
These breakthroughs are not just academic; they have the potential to revolutionize healthcare, agriculture, and biotechnology. However, they also raise important questions about bioethics, regulation, and the social implications of such powerful tools.
Through this exploration of DNA's structure and function, we gain not only insight into the mechanics of life itself but also a deeper understanding of the responsibilities that come with such profound knowledge.
Intro to DNA
Understanding DNA is like trying to grasp the very fabric of life itself. This article aims to unfold the complexity of DNA molecules, detailing not only their structure but the myriad functions underpinning various biological processes. DNA, or deoxyribonucleic acid, serves as the blueprint for all living organisms, influencing everything from physical traits to susceptibility to certain diseases. The exploration of this molecule is not just an academic journey but a gateway to grasping how life works at its most fundamental level.
Historical Background of DNA Discovery
The quest to comprehend DNA began in the aftermath of the 19th century. In 1869, Friedrich Miescher, a Swiss biochemist, stumbled upon a substance while examining cell nuclei in pus. He coined this substance "nuclein"—the first step towards identifying DNA. Fast forward to the early 20th century, scientists like Avery, MacLeod, and McCarty began to suspect that nucleic acids were the carriers of genetic information.
However, the watershed moment came in 1953 when James Watson and Francis Crick proposed their model of the DNA double helix, propelled by previous work of other scientists, including Rosalind Franklin and Maurice Wilkins. This model explained how genetic information is stored and transmitted across generations. Their discovery not only cemented the role of DNA in heredity but also opened the floodgates for molecular biology as a discipline.
Importance of DNA in Modern Science
Today, DNA plays a pivotal role in various scientific fields, from genetics to medicine and biotechnology. Its implications are extensive:
- Genetics: DNA is central to understanding inheritance patterns and genetic disorders. By unraveling the genetic code, we can identify gene mutations that lead to diseases.
- Medicine: Techniques like genetic screening and personalized medicine use DNA to tailor healthcare to individual needs, shifting the paradigm from a one-size-fits-all approach.
- Forensics: DNA profiling has revolutionized criminal investigations, providing definitive evidence that can identify or exclude suspects based on genetic material found at crime scenes.
- Agriculture: Genetic modification of crops ensures better resilience against pests and environmental stressors, enhancing food security.
The capability to analyze and manipulate DNA has made it a cornerstone in various scientific explorations, allowing researchers to probe into the mysteries of life while also raising critical ethical questions. The intricate dance of nucleotides forms a story—one that continues to unfold with every new technological advance and discovery.
Chemical Composition of DNA
The study of DNA's chemical composition is indispensable when discussing its structure and function. Understanding the components that make up this complex molecule helps unravel the intricate processes underpinning life itself. Each element of the DNA molecule contributes significantly not just to its stability but also to its function in heredity and cellular activities. Dissecting the chemical structure affords insights into how genetic information is stored and transferred, an essential foundation for fields ranging from genetics to biotechnology.
Nucleotides: The Building Blocks
Nucleotides are the fundamental units of DNA, resembling the bricks that construct a sturdy house. Each nucleotide comprises three core parts: a phosphate group, a sugar molecule, and a nitrogenous base. The arrangement and connection of these building blocks define the specific sequences of DNA.
- Phosphate Group: This part of the nucleotide carries a negative charge and is responsible for linking the sugar molecules in the backbone of the DNA strand. It imparts an essential structural integrity, ensuring that the DNA maintains its double helical form.
- Sugar Molecule: In DNA, the sugar is deoxyribose, which lacks one oxygen atom compared to the ribose sugar found in RNA. This subtle difference is pivotal, influencing the stability and structure of DNA.
- Nitrogenous Base: Here lies the key to genetic variability. The four types of nitrogenous bases—adenine, thymine, cytosine, and guanine—combine in myriad sequences to encode the genetic instructions of an organism.
"The unique sequences of nucleotides are what make each DNA strand a distinct blueprint, catering to the individual traits of every living being."
"The unique sequences of nucleotides are what make each DNA strand a distinct blueprint, catering to the individual traits of every living being."
Sugar-Phosphate Backbone
The sugar-phosphate backbone serves as the backbone, both structurally and conceptually, of DNA strands. It consists of alternating sugar and phosphate groups linked through phosphodiester bonds. These bonds not only provide a scaffold that physically supports the nitrogenous bases but also ensure stability under biological conditions.
The configuration of this backbone allows DNA to twist into a helix, protecting the more reactive bases from damage. The rigidity brought by this backbone is crucial. It helps DNA resist various environmental factors that could otherwise cause mutations or damage, which is vital for maintaining genetic integrity.
Nitrogenous Bases: Adenine, Thymine, Cytosine, Guanine
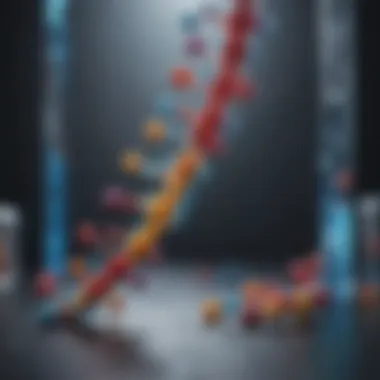
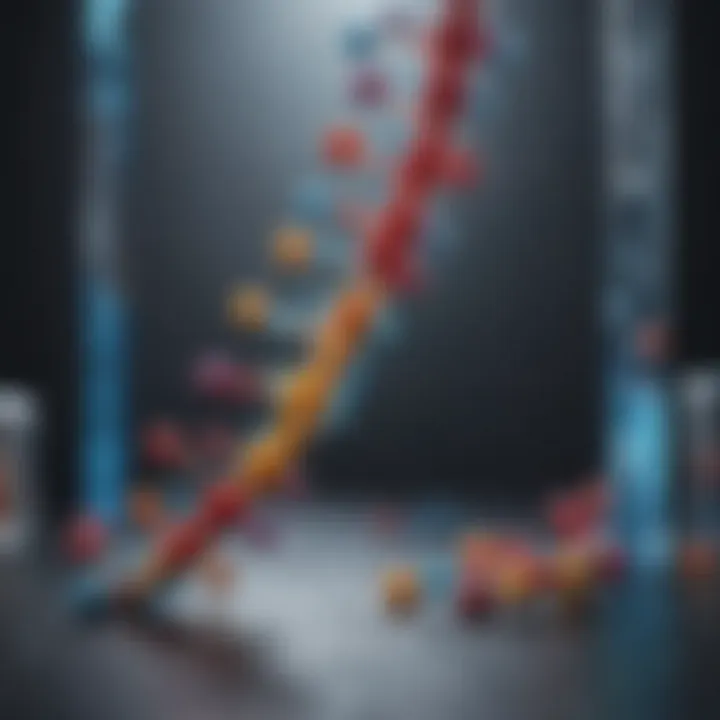
The real magic of DNA lies in its nitrogenous bases. Adenine pairs with thymine, while cytosine pairs with guanine. This specific pairing is known as complementary base pairing and is governed by hydrogen bonds; adenine and thymine form two hydrogen bonds, while cytosine and guanine form three.
These bases are not just crucial for forming the genetic code; their sequences dictate the formation of proteins, which carry out most cellular functions. Here’s a brief overview of each:
- Adenine (A): Often involved in energy transfer and can also act in signaling pathways.
- Thymine (T): Unique to DNA, thymine plays a pivotal role in stabilizing the structure of the DNA double helix.
- Cytosine (C): It not only pairs with guanine but is also involved in regulating gene expression.
- Guanine (G): This base is crucial in the synthesis of important biomolecules like ATP, GTP, and cGMP.
A deeper knowledge of these nitrogenous bases can enhance our understanding of mutations and their potential impact on cellular processes. By modifying these sequences, scientists can influence gene expression and bring forth various innovations in genetic research.
Double Helix Structure
The double helix structure of DNA is not just a beautiful twist of fate; it serves as the very foundation of genetic information storage and transmission. This configuration is pivotal for several reasons. Firstly, its unique shape allows for the secure packaging of genetic material within the nucleus of cells, while also providing a mechanism for accurate replication during cell division. This structural choice is crucial, as the fidelity of genetic information passed from one generation to the next is fundamentally reliant on this architectural design.
Watson and Crick Model
The Watson and Crick model, proposed in 1953, was a watershed moment in molecular biology. James Watson and Francis Crick's insights into the double helix not only elucidated the structure of DNA but also hinted at its function. Their model depicted two strands of nucleotides that coil around each other, resembling a twisted ladder. The rungs of this ladder are formed by pairs of nitrogenous bases—adenine pairs with thymine, and cytosine pairs with guanine. This complementary base pairing is essential, as it allows for precise replication of DNA during cell division, ensuring that genetic information remains intact.
Moreover, Watson and Crick’s model highlighted the significance of hydrogen bonds between these base pairs. Such bonds, although weak individually, collectively ensure that the strands are tightly held together, which is critical for both the stability and integrity of genetic material.
"The beauty of the double helix model is not just in its aesthetics but in its ingenious solution to the problem of genetic replication."
"The beauty of the double helix model is not just in its aesthetics but in its ingenious solution to the problem of genetic replication."
Antiparallel Strands
One of the most interesting features of the double helix is that its strands run in opposite directions, known as antiparallel orientation. This means that if one strand runs from the 5’ end to the 3’ end, the opposite strand will run from the 3’ end to the 5’ end. This arrangement is key for several reasons. It provides enzymes that replicate and read DNA with critical directional cues. DNA polymerase, for instance, can only add new nucleotides in one direction, which is 5’ to 3’. Therefore, the antiparallel nature of DNA ensures that both strands can be replicated simultaneously, albeit using different processes for the leading and lagging strands.
Base Pairing Rules
Base pairing rules are foundational to understanding how DNA encodes genetic information. As established by Watson and Crick, adenine always pairs with thymine, while cytosine pairs with guanine. This specific pairing is enforced through hydrogen bonds that offer just the right stability yet allow for the slight flexibility needed during replication and transcription.
This meticulous pairing does more than just support DNA structure; it assists in the accurate copying of sequences and thereby influences gene expression. Any mutations or errors in base pairing can lead to significant consequences for the organism, manifesting in various genetic disorders or cancers. Understanding these rules allows researchers to predict how changes in one strand may affect the complementary strand and, in turn, the overall function of DNA.
In summary, the double helix structure, with its historical significance and practical implications in genetics, remains a subject of endless fascination and rigorous study.
DNA Replication Process
Understanding DNA replication is like piecing together the blueprint of life itself. This process is crucial because it ensures that genetic information is accurately transmitted during cell division. Every living organism, whether it be as simple as a bacteria or as complex as a human, relies on the fidelity of this process to function properly. Errors in replication can lead to mutations, which can have a spectrum of effects, from benign to detrimental. Beyond mere replication, this section also delves into roles of enzymes, structural considerations, and the significance of leading and lagging strands.
Mechanism of DNA Replication
At the core of DNA replication lies a beautifully orchestrated series of events. The process begins with the unwinding of the double helix, a task performed by specialized proteins. As the strands separate, each becomes a template for the synthesis of a new partner strand. This is not a simple task; it requires precision and coordination. After the strands are unwound, short segments of RNA, known as primers, are laid down on the template strands to give DNA polymerases the necessary starting point. It’s like having a starting line for a race; without it, you simply can't take off.
The synthesis occurs continuously on one of the strands – termed the leading strand – while the other, the lagging strand, is synthesized in smaller, discontinuous segments called Okazaki fragments. Once the segments are formed, they need to be stitched together, which is where DNA ligase comes into the picture. The entire mechanism of DNA replication showcases the brilliance of cellular machinery and the high stakes involved in maintaining genetic integrity.
Role of Enzymes in Replication
The process of DNA replication is dependent on several key enzymes, each playing a specific role to ensure smooth operation. Without these enzymes, replication would be a disorganized jumble, making accurate genetic information transfer nearly impossible.
DNA Polymerase
DNA polymerase is pivotal to the replication process. This enzyme adds nucleotides to the growing DNA strand, matching them precisely to the template strand. One of the key characteristics of DNA polymerase is its ability to proofread its work. It checks if the last nucleotide added is correctly paired, which significantly reduces the error rate during DNA replication. The unique feature of DNA polymerase is its speed; it can add around 1,000 nucleotides to a growing chain every second in human cells. This efficiency is vital for quick cell division, especially in rapidly growing tissues. However, it has its limitations, functioning optimally only in specific circumstances, such as needing the presence of a primer to begin synthesis.
Helicase
Helicase plays an equally important role in this dance of enzymes. It unwinds the DNA double helix, separating the strands so they can each serve as templates. The key characteristic of helicase is its energy requirement; it utilizes the energy from ATP hydrolysis to function, essentially powering its movement along the DNA strand. The unique aspect of helicase is its ability to unwind DNA at a rapid pace—up to 1,000 base pairs per second! However, this speed can be a double-edged sword; if helicase works too quickly, it can create tangles and other structural issues in the DNA.
Ligase
Lastly, we have DNA ligase, the unsung hero of replication. After Okazaki fragments on the lagging strand are synthesized, ligase comes in to connect these fragments, forming a continuous strand. The primary distinguishing character of ligase is its role in sealing nicks in the DNA. Its function is critical for maintaining the integrity of the DNA molecule after replication. A unique feature of DNA ligase is its ability to also participate in DNA repair processes, thus showcasing its versatility. Though widespread in nature, it can be challenged by complex repair pathways, especially when lesions or errors occur outside of standard replication processes.
Leading and Lagging Strands
The concepts of leading and lagging strands often give students a run for their money. After all, why can’t both strands be synthesized in the same continuous manner? The answer lies in the antiparallel nature of DNA. The leading strand is synthesized smoothly and continuously toward the replication fork, while the lagging strand must be synthesized in short bursts away from the fork. This staggered synthesis necessitates the action of multiple enzymes and mechanisms working in concert. Understanding these strands is critical because it highlights how even minor differences in molecular behavior can have large implications for cellular processes.
The intricate balance of enzyme activity and strand synthesis during DNA replication is a testimony to nature's precision engineering.
The intricate balance of enzyme activity and strand synthesis during DNA replication is a testimony to nature's precision engineering.
As we wrap up this section, it’s clear that the DNA replication process is foundational not just for biology as we know it, but for the existence of life itself. With each replication cycle, the cell perpetuates its genetic legacy, underscores the roles of specific enzymes, and enforces the structural integrity of its genetic blueprint.
Gene Expression and Regulation
Gene expression and regulation represent crucial processes in cellular biology. They are fundamental to how an organism translates its genetic information into functional products, such as proteins. This section will illuminate the roles of transcription, translation, and various regulatory elements that finely tune these processes.
Transcription Process
Transcription is the initial phase of gene expression. In this process, the DNA sequence of a gene is copied into messenger RNA (mRNA). This step begins when the enzyme RNA polymerase binds to the promoter region of a gene. The promoter serves as a signal for the machinery, guiding it to the right starting point on the DNA strand. Once bound, RNA polymerase unwinds the DNA double helix and assembles nucleotides to form an RNA transcript.
After the completion of transcription, the mRNA undergoes several modifications. This includes capping at the 5' end and polyadenylation at the 3' end, which protect the molecule and aid in the transportation out of the nucleus. The mRNA then leaves the nucleus, entering the cytoplasm where it can be translated into a protein.
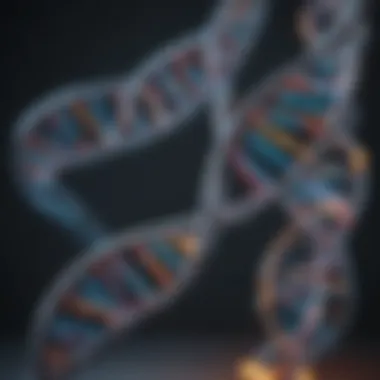
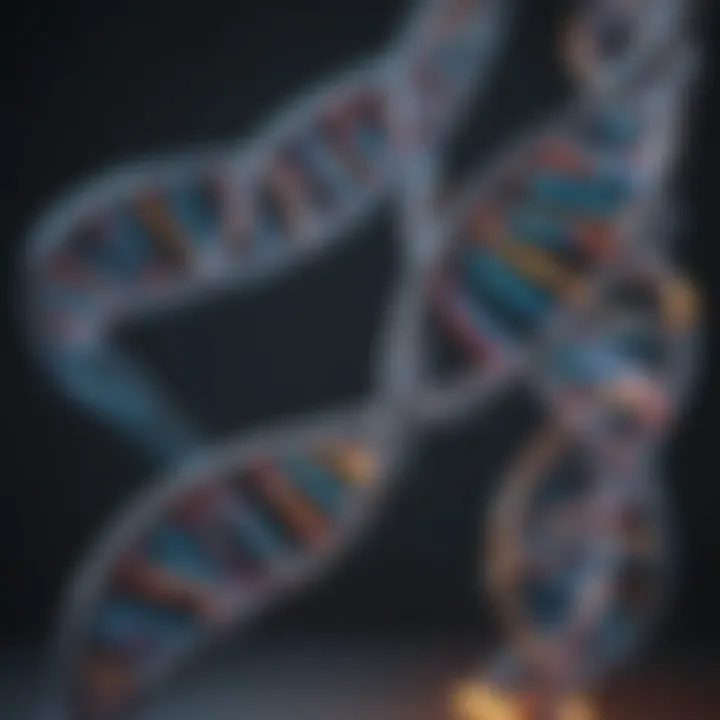
Translation and Protein Synthesis
Translation is the second phase of gene expression, occurring in the cytoplasm. This process translates the genetic code carried by mRNA into a sequence of amino acids, ultimately forming a protein. Ribosomes, composed of rRNA and proteins, are the sites where translation happens. Each ribosome reads the mRNA in sets of three bases known as codons, each corresponding to a specific amino acid.
Transfer RNA (tRNA) molecules play a crucial role here; they transport the appropriate amino acids to the ribosome and match their anticodons to the codons on the mRNA. This intricate interplay continues until a stop codon is reached, at which point the newly synthesized protein is released into the cell, ready to perform its function.
Regulatory Elements of Genes
Gene expression does not simply happen; it is tightly regulated by several key components.
Promoters
Promoters are critical sequences located at the start of a gene, where transcription initiates. They determine the binding site for RNA polymerase and other transcription factors necessary to kickstart the process. One key characteristic of promoters is their ability to contain specific sequences that can enhance or inhibit transcription efficiency. The presence of a strong promoter ensures a high level of gene activity, while weak promoters lead to reduced expression. The versatility of promoters makes them a favorable focus for understanding gene regulation.
Enhancers
Enhancers are sequences that can significantly boost the transcriptional activity of associated genes. They can be located far from the promoter and can function regardless of their orientation. One noteworthy aspect of enhancers is the binding of various transcription factors, which can interact with the promoter region through DNA looping. This interaction often leads to substantial increases in gene expression, highlighting their importance in fine-tuning cellular responses.
Silencers
Silencers are sequences that actively inhibit gene expression. These elements can bind to repressor proteins, which in turn block transcription by preventing the binding of RNA polymerase to the promoter. A distinctive characteristic of silencers is their flexible positioning, allowing them to be located upstream or downstream from the genes they regulate. Understanding the dual roles of both enhancers and silencers provides a nuanced picture of how genes can be expressed or silenced as needed, which is pivotal in various biological processes including development and response to environmental changes.
Gene expression and regulation are pivotal for maintaining cellular functions and behaviors, reflecting the underlying genetic blueprint of an organism.
Gene expression and regulation are pivotal for maintaining cellular functions and behaviors, reflecting the underlying genetic blueprint of an organism.
Mutations and Their Consequences
Understanding mutations is fundamental in grasping the complexity of genetics. These changes, whether deliberate or accidental, can significantly affect an organism's traits and functions. In this section, we explore various types of mutations, their ramifications, and their effects on protein synthesis and overall biology. By examining mutations closely, we can identify their significance in evolution, adaptation, and even in the field of medicine.
Types of Mutations
When it comes to mutations, there are different flavors, each with distinct characteristics and implications for DNA and protein function. Here’s a closer look at the three primary types of mutations:
Point Mutations
Point mutations refer to the alterations of a single nucleotide base in the DNA sequence. This can happen through a substitution, wherein one base is replaced by another. This specific kind of mutation holds particular relevance due to its potential to directly alter protein sequences. One key aspect is the fact that point mutations can be silent, missense, or nonsense, each leading to different outcomes in gene expression and functionality. It stands out as a popular subject in genetic studies because it often leads to diseases, such as sickle cell anemia, when it results in a significant change in the protein's function. The unique feature here is its subtlety, as it might not always produce observable changes in the organism. However, when it does, the consequences can be profoundly beneficial or severely detrimental.
Insertions and Deletions
Insertions and deletions (collectively termed indels) involve the addition or removal of one or more nucleotide bases. These are among the most disruptive types of mutations, as they can shift the reading frame of the gene, drastically altering the downstream amino acid sequence. This makes them critical for the study of gene functionality. They are often highlighted for their potential to lead to frameshifts, which can render proteins nonfunctional. The unique aspect of indels is their ability to cause larger-scale changes than simple point mutations, underscoring the importance of maintaining genetic integrity. While they can lead to novel traits advantageous for survival, they can equally result in dire consequences, such as genetic disorders.
Chromosomal Mutations
Chromosomal mutations are larger-scale alterations that can affect the structure and number of chromosomes. They can include duplications, inversions, deletions, or translocations. The scale of these mutations makes them particularly impactful, hence their notable place in genetic discussions. These mutations can lead to significant changes in gene dosage and expression, which can in turn lead to serious consequences during cell division. The unique characteristic here lies in their ability to impact multiple genes simultaneously, thus having cascading effects on an organism’s biology. Often a double-edged sword, chromosomal mutations can be a source of genetic diversity, however they can also lead to various cancers and genetic syndromes.
Impact on Protein Function
The repercussions of mutations extend beyond genetic sequences; they resonate with protein functionality as well. Mutations can tweak the amino acid sequence of proteins, sometimes subtly, other times dramatically. Such changes may impact protein folding, stability and overall activity.
To summarize, the influences of mutations are multifaceted, with potential outcomes ranging from adaptations that benefit survival, to malfunctions that lead to disease. The study of mutations remains an essential pursuit in understanding the genetic framework of life.
"Mutations are the essence of evolution, but they can also be the roots of diseases."
"Mutations are the essence of evolution, but they can also be the roots of diseases."
Whether it's through enhancing our grasp of genetics or developing therapies for genetic disorders, recognizing the broad implications of mutations is pivotal for future research and applications.
Current Advances in DNA Research
Understanding DNA research is akin to navigating a vast labyrinth, with every turn revealing new insights and implications for science and society. The progress in this field is not just about deciphering genetic codes but also about reshaping medical practices, enhancing agricultural productivity, and potentially altering ecosystems. This section examines the latest developments, notably in CRISPR technology, genomic sequencing, and their applications in medicine and biotechnology. Each facet contributes a unique perspective to how we perceive life and its possibilities.
CRISPR and Genetic Editing
CRISPR technology has become synonymous with genetic editing, representing a breakthrough that can be imagined as a biological word processor of sorts, allowing scientists to rewrite the language of life. It is a tool derived from the immune systems of bacteria, which they use to defend against viruses. This natural mechanism has been co-opted for editing genes with remarkable precision and efficiency.
CRISPR’s key advantages include:
- Cost-effectiveness: Compared to previous editing techniques, CRISPR is significantly cheaper and more accessible to researchers.
- Ease of use: With straightforward protocols, scientists can implement CRISPR in diverse organisms, leading to rapid iterations in research.
- Versatility: CRISPR can target multiple genes simultaneously, opening up the door for more complex modifications.
- Precision: The ability to make precise edits reduces the likelihood of off-target effects, which can lead to unintended consequences in genetic research.
However, this powerful capability does not come without ethical considerations. The potential to edit human embryos, for instance, raises questions about the implications for future generations and the moral standing of designing genetic traits. This ongoing ethical debate necessitates careful guidance as society grapples with the realities of genetic superiority and the consequences of 'playing god'.
"The power of CRISPR technology is a double-edged sword; its precision offers vast potential, yet its applications challenge us to think about the ethics of altering life itself."
"The power of CRISPR technology is a double-edged sword; its precision offers vast potential, yet its applications challenge us to think about the ethics of altering life itself."
Genomic Sequencing Technologies
The landscape of genomic sequencing has undergone radical transformation, much like the evolution of communication from letters to instant messaging. High-throughput sequencing technologies have propelled us into an era where entire genomes can be sequenced in a matter of days or even hours, making this process more feasible than ever before.
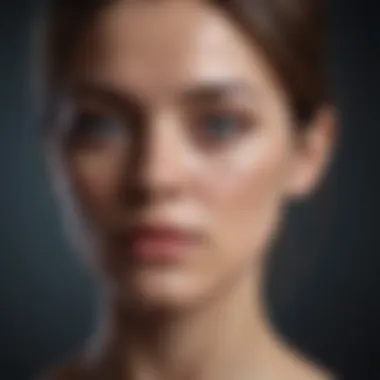
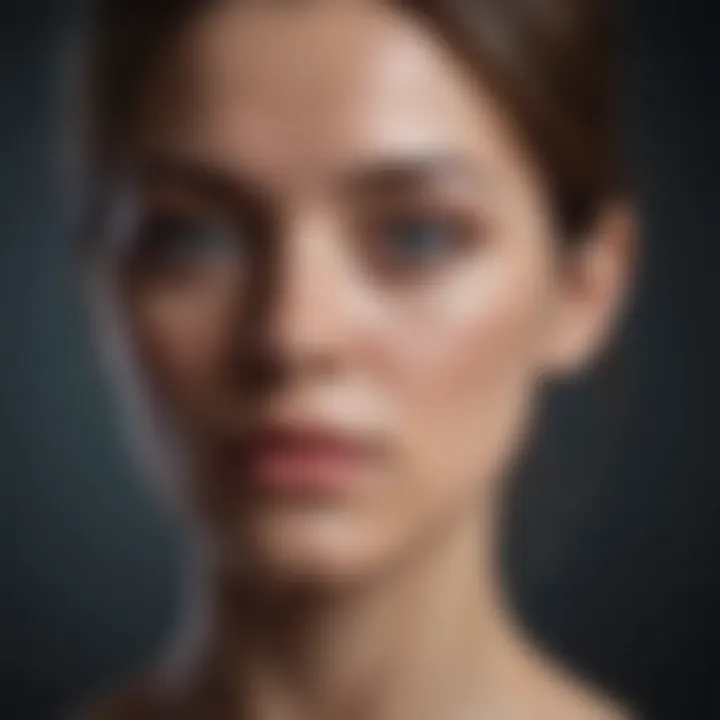
Some emerging technologies include:
- Next-Generation Sequencing (NGS): This method allows for massively parallel sequencing, significantly boosting the quantity and speed of data generation.
- Single-Cell Sequencing: This approach facilitates the analysis of the genomic makeup of individual cells, blending resolution with accuracy and unveiling cellular heterogeneity previously masked in bulk analysis.
- Long-Read Sequencing: Technologies such as those developed by Pacific Biosciences and Oxford Nanopore drastically improve the assembly of complex genomes by generating longer DNA reads, helping to resolve repetitive regions that can stymie shorter-read technologies.
These advancements collectively enhance our understanding of genetic variations, contributing to personalized medicine and the ongoing quest to pinpoint the genetic underpinnings of diseases ranging from cystic fibrosis to cancer. Furthermore, they enable precise population analyses, vital for understanding evolution and biodiversity.
Applications in Medicine and Biotechnology
The implications of current advances in DNA research ripple through the fabric of medicine and biotechnology, bridging the gap between theoretical science and real-world applications. These developments are not just academic; they are poised to revolutionize healthcare and agricultural practices.
Key applications include:
- Targeted therapies: Cancer treatments tailored to the genetic profile of a tumor employ gene therapies that specifically target the molecular mechanisms driving the disease.
- Gene therapy: Conditions caused by mutations, such as muscular dystrophy and hemophilia, are beginning to be treated by repairing or replacing defective genes in patients.
- Synthetic biology: This field applies genetic principles to design and construct new biological parts, devices, and systems, leading to innovations such as biofuels and biodegradable plastics.
- Crop modification: Genetic modifications enhance food security through resistant crops that tolerate pests and environmental stressors, directly contributing to sustainable agriculture.
In summary, the advances in DNA research extend beyond pure science; they infuse ethical quandaries and societal questions. As technology progresses, fostering a dialogue around responsible usage and ethical standards will be crucial. Each step forward propels humanity into a future shaped by our choices in genetic science.
Ethical Considerations in DNA Research
In recent years, discussions around DNA research have become increasingly significant, reflecting society's evolving relationship with genetic science. The advent of technologies such as CRISPR and advances in genomic sequencing have opened up vast possibilities, yet they also raise profound ethical dilemmas that demand thorough consideration. Addressing these ethical issues isn't just a scholarly exercise; it pertains to societal values, individual rights, and the broader implications of manipulating life at a molecular level.
Gene Editing Ethics
Gene editing, particularly through techniques like CRISPR, has stirred a pot of ethical debates that can be as complicated as the science it stands for. One major concern is the potential for designer babies, or genetically modified individuals who are engineered for specific traits. While this notion may appear distant, advancements are moving at such an astonishing pace that it becomes an imminent reality.
Some specific ethical concerns in gene editing include:
- Equity in Access: There's a tangible risk that only those with considerable resources may benefit from these technologies. If gene editing remains predominantly in the hands of a wealthy few, it could lead to a society divided by genetic privilege.
- Informed Consent: Editing genes of embryos involves making irreversible decisions for individuals who cannot voice their own consent. This raises questions about the autonomy of future generations.
- Consequences of Off-Target Effects: Gene editing isn't foolproof. It can produce unintended genetic changes, which may have unforeseen consequences. Hence, determining accountability for possible negative outcomes remains a complex issue yet to be resolved.
Implications for Genetic Privacy
As DNA research expands and becomes more accessible, concerns over genetic privacy also heighten. The ability to analyze genetic information means that an individual’s genetic predispositions and potential health risks can be discerned and misused. The implications extend beyond the personal to societal norms, affecting how individuals perceive their identities.
Consider the following aspects of genetic privacy:
- Data Ownership: Who owns genetic data? Should individuals retain rights over their own genetic information once it gets shared with repositories or research institutes? This question invites discussions that cross legal, ethical, and societal boundaries.
- Potential Discrimination: Employers or insurance companies could misuse genetic information to discriminate, either by favoring those with 'desirable' traits or penalizing individuals based on genetic vulnerabilities. Such practices underline the importance of robust legal protections against genetic discrimination.
- New Norms of Privacy: As more people consent to genetic testing for health risks, a cultural shift may arise regarding privacy. People might become comfortable sharing intimate details of their genetic makeup, raising questions about the limits of personal privacy as society becomes increasingly intertwined with genetic information.
"The essence of ethics in DNA research isn't just about what we can do; it's fundamentally about what we should do."
"The essence of ethics in DNA research isn't just about what we can do; it's fundamentally about what we should do."
Navigating these ethical landscapes demands not only rigorous scientific inquiry but also an active dialogue among ethicists, scientists, and the broader community. Understanding these implications is critical for all stakeholders in DNA research.
Future Directions in DNA Research
The exploration of DNA is far from being a finished chapter in biological science. As researchers continue to peel back the layers of this complex molecule, we find ourselves positioned at the nexus of remarkable advancements and ethical quandaries. Future directions in DNA research hold significance not only for understanding the molecular bases of life but also for implicating broader societal concerns. The trajectory here is not solely about scientific inquiry but also about harnessing technology for improved health outcomes and addressing the ramifications that come with such capabilities.
Emerging Technologies and Techniques
In recent years, several technologies have emerged that could redefine how we utilize DNA-related knowledge. One such technology is nanopore sequencing, which enables the real-time analysis of long DNA strands. Unlike traditional methods, this approach allows scientists to see the sequence of bases as they pass through a tiny pore, facilitating the identification of complex genetic variants. This technology could transform personalized medicine, allowing for quicker diagnosis and tailored treatments based on an individual's genetic makeup.
Another transformative technique is single-cell sequencing, which permits researchers to analyze the genetic material of individual cells. This fine-grained approach is invaluable in fields such as cancer research, where the tumor microenvironment can significantly affect therapeutic outcomes. By understanding the genetic landscape of each cell, scientists can develop strategies to target specific subpopulations within a tumor, potentially improving treatment efficacy.
"As we stand on the brink of a genomic revolution, the need for responsible implementation and understanding the underlying ethical stakes cannot be overlooked."
"As we stand on the brink of a genomic revolution, the need for responsible implementation and understanding the underlying ethical stakes cannot be overlooked."
Moreover, synthetic biology is paving the way for various innovative applications, such as engineering organisms to produce pharmaceuticals or biofuels. Researchers are devising new ways to design and construct biological parts, devices, and systems. This could lead to sustainable production methods that lessen our reliance on fossil fuels or allow for the creation of custom microorganisms that target specific diseases.
Potential for Disease Prevention
As we look ahead, the potential of DNA research in disease prevention becomes increasingly palpable. The convergence of genome-editing tools, particularly CRISPR-Cas9, has unlocked new avenues for addressing genetic disorders at their source. For instance, researchers are putting forth considerable effort into using CRISPR technology to modify genes that cause conditions like sickle cell anemia and certain types of muscular dystrophy. This approach could pave the way for revolutionary treatments that not only manage symptoms but eradicate the root cause.
Furthermore, leveraging advances like RNA interference could streamline the development of therapies against viral infections. By silencing the genes vital for a virus's replication, we limit its ability to propagate within the host. This strategy has already shown promise in preliminary studies targeting viruses such as HIV and hepatitis B.
The implications extend into preventative medicine, where understanding an individual's genetic predisposition to various diseases can lead to proactive health measures. Genetic screenings can provide insights into one's risks for conditions like heart disease or diabetes, prompting preemptive lifestyle or medical interventions. This proactive stance could shift our healthcare system from one that reacts to disease to one that actively prevents it.
As we venture into these promising areas of research, it’s imperative to keep a keen eye on ethical implications. The ability to modify genetic structures brings forth questions about consent, the scope of interventions, and potential long-term consequences. Thus, the dialogue surrounding future directions in DNA research must include not just the scientific community but also reach broader societal stakeholders. This ensures that advancements in DNA research benefit humanity while navigating the complex moral landscape it presents.
Closure
In closing, the exploration of DNA's structure and function is not just an academic endeavor; it represents a cornerstone of biological sciences and genetics. This article has journeyed through various facets of DNA, discussing its chemical composition, structural features, replication processes, and gene expression. Each element has its own distinct significance, weaving a complex narrative that illustrates not only how genetic information is stored and expressed but also how it can be manipulated for advancements in science and medicine.
Summary of Key Points
To encapsulate the crucial themes discussed:
- The chemical composition of DNA is fundamentally based on nucleotides, which are the building blocks made up of a sugar, phosphate group, and nitrogenous bases.
- Understanding the double helix structure created by Watson and Crick is essential to grasp the concepts of genetic replication and expression.
- The DNA replication process showcases the detailed roles of enzymes like DNA polymerase and helicase, essential for accurate genetic copying.
- Gene expression encompasses transcription and translation, translating genetic codes into functional proteins, pivotal for life.
- Various mutations can lead to significant changes in protein function, impacting health and development.
- The emergence of CRISPR technology and genomic sequencing has opened doors for precision in genetic engineering, with profound implications for treatments and disease management.
- Ethical considerations highlight the responsibility that comes with powerful genetic technologies, urging caution and depth in discussions surrounding genetic privacy and gene editing.
Significance of Ongoing Research
Continuous research in DNA is of utmost importance for multiple reasons:
- It fuels innovation in medical treatments, leading to potential cures for genetic disorders and personalized medicine strategies.
- Understanding how DNA functions can lead to insights about evolution, biodiversity, and the intricate workings of ecosystems.
- Ongoing projects in the realm of synthetic biology could revolutionize how we approach bioengineering, leading to microorganisms that could produce biofuels or pharmaceuticals.
- The exploration also has societal implications, pushing us to confront ethical questions about genetic manipulation and the future of humanity.
As we step into an era marked by rapid advancements in genetic research, the continuous quest to untangle the complexities of DNA is paramount. The foundations laid by past discoveries will guide future explorations, ensuring DNA remains a focal point in the journey of scientific inquiry and technological development.